Recent Understanding of the Molecular Mechanisms of Alzheimer's Disease
Marcus OW Grimm1,2,3* and Tobias Hartmann1,2,3
1Experimental Neurology, Saarland University, Kirrbergerstrasse, 66421 Homburg/Saar, Germany
2Deutsches Institut für DemenzPrävention (DIDP), Saarland University, Kirrbergerstrasse, 66421 Homburg/Saar, Germany
3Neurodegeneration and Neurobiology, University, Kirrbergerstrasse, 66421 Homburg/Saar, Germany
- *Corresponding Author:
- Marcus OW Grimm
Saarland University, Kirrbergerstrasse
66421 Homburg/Saar, Germany
Tel: +49-6841-1647919
Fax: +49-6841-1647801
E-mail: marcus.grimm@uks.eu
Received December 08, 2011; Accepted January 18, 2012; Published January 22, 2012
Citation: OW Grimm M, Hartmann T (2012) Recent Understanding of the Molecular Mechanisms of Alzheimer’s Disease. J Addict Res Ther S5:004. doi:10.4172/2155-6105.S5-004
Copyright: © 2012 OW Grimm M, et al. This is an open-access article distributed under the terms of the Creative Commons Attribution License, which permits unrestricted use, distribution, and reproduction in any medium, provided the original author and source are credited.
Visit for more related articles at Journal of Addiction Research & Therapy
Abstract
Alzheimer’s disease (AD) is the most common neurodegenerative disorder in the aged population. Pathological hallmarks of AD are the presence of extracellular senile plaques and intracellular neurofibrillary tangles. Extracellular plaques consist of aggregated amyloid-β (Aβ) peptides derived from sequential proteolytic cleavage of the amyloid precursor protein (APP) by β- and γ-secretase. Neurofibrillary tangles are composed of a hyperphosphorylated form of the microtubule-associated protein tau. This review summarizes the current understanding in the molecular mechanisms leading to Aβ generation as well as hyperphosphorylation of tau and the mechanisms of Aβ-induced neurotoxicity including Ca2+ dyshomeostasis, mitochondrial dysfunction, increased oxidative stress, cholinergic dysfunction and neuroinflammation finally resulting in neuronal/synaptic dysfunction and neuronal loss.
Alzheimer’s Disease – Epidemiology and Pathological Hallmarks
Alzheimer’s disease (AD) is the most common form of dementia in the elderly and is characterized in patients by an inexorably progression, causing problems with memory, thinking and behaviour. Currently 25 million people worldwide are estimated to be affected by AD with the highest number in North America, Latin America, Western Europe, China and the western pacific [1]. Epidemiological studies predict that the incidence of AD will increase due to increasing life expectancy to 42.3 million in 2020 and 81.1 million in 2040 [1,2], emphasizing AD as a major public health concern.
The two main histopathological hallmarks of AD are extracellular amyloid plaques and intracellular neurofibrillary tangles in vulnerable brain regions like hippocampus and cortex [3]. The amyloid plaques, also called senile plaques, are mainly composed of amyloid-beta peptides (Aβ), most of which are 38 to 43 amino acids long [4]. Aβ peptides are generated by sequential processing of the amyloid precursor protein (APP), a large type-I transmembrane protein [5] that belongs to an evolutionary conserved protein family including beside APP, the APP-like protein 1 (APLP1) and 2 (APLP2) in mammals [6,7] with the Aβ domain to be unique to APP [8]. The second characteristic pathological hallmark of AD, the intracellular neurofibrillary tangles, consist of an abnormally phosphorylated form of the microtubule-associated protein tau [9]. In contrast to amyloid plaques, which accumulate extracellularly in the brains of AD affected individuals and have been long believed to cause neuronal damage and cell death, neurofibrillary tangles are found inside neurons and their branching points like axons and dendrites [10,11].
Proteolytic Processing of APP
Amyloidogenic APP processing
Proteolytic processing of APP leading to Aβ peptides is a ubiquitous cellular process involving β- and γ-secretase activity (Figure 1). The first step in Aβ production is APP cleavage by β-secretase, generating the N-terminus of Aβ. BACE 1 (β-site APP cleaving enzyme, also called Asp2 or memapsin2) was identified as the major β-secretase, a membrane-bound protease that belongs to the pepsin family of aspartyl proteases [12-14]. Aβ generation is abolished in cultures of BACE1-deficient embryonic cortical neurons [15] and in BACE1 knock-out mice [16,17]. Whereas Luo and Roberdset al. [16,17] reported a normal phenotype of BACE1-deficient mice, more recent studies found severe phenotypic abnormalities in BACE1 knock-out mice [18], challenging BACE1 as safe drug target for AD. Furthermore, additional BACE1 substrates have been reported, suggesting a variety of BACE1 physiological functions. Identified substrates include proteins with important neuronal function, like the β2-subunit of the voltage-gated sodium channel (Navβ2) [19,20], important for normal action potential regulation and neuronal excitability, and neuregulin-1 [21,22], as ligand for members of the ErbB family of receptor-tyrosine kinases, involved in synapse formation, myelination of central and peripheral axons and the regulation of neurotransmitter expression and function [23,24]. Recently, Kim et al. [25] reported that endogenous BACE1 activity also regulates total and surface levels of voltage-gated sodium channels in mouse brains, which also suggests that therapeutic inhibition of BACE1 activity may affect neuronal membrane excitability in AD patients. Notably, soluble β-secreted APP (sAPPβ), representing the N-terminal domain of APP that is cleaved off by BACE1, has been shown to function as a death receptor 6 ligand and mediates axonal pruning and neuronal cell death [26].
BACE1 is highly expressed in neurons, whereas the homolog BACE2 is expressed at very low levels in most brain regions [27] and has not the same cleavage activity on APP as BACE1 [28], emphasizing that BACE1 is the primary β-secretase. However, a potential contribution of BACE2 in amyloidogenic processing and AD pathogenesis cannot be excluded. BACE1 requires an acidic environment for optimal enzyme activity and is therefore mainly found in intracellular compartments with a low pH like endosomes and late Golgi-compartments [13,29,30]. Additionally, BACE1 can be found at the cell surface [13,29,31,32] and recycles between the cell surface and endosomal compartments [29,31]. Beside BACE1 and BACE2, cathepsin B is discussed as an additional β-secretase as inhibition of cathepsin B has been found to reduce Aβ production [33,34]. However, recent findings also implicate cathepsin B as an Aβdegrading enzyme in respect of AD [35,36].
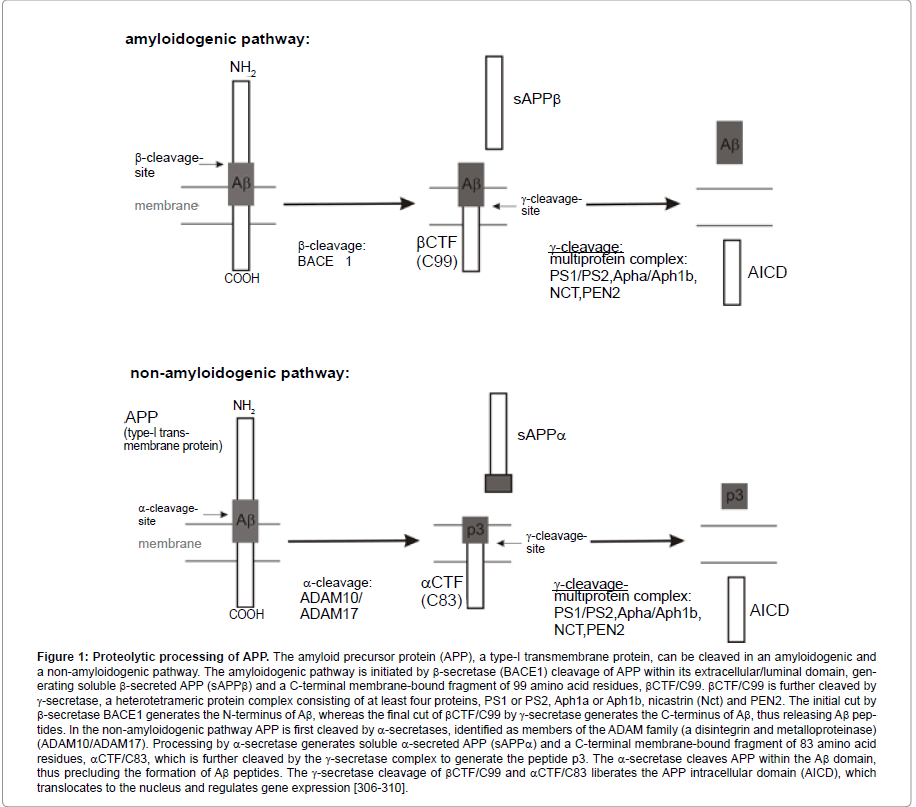
Figure 1:Proteolytic processing of APP. The amyloid precursor protein (APP), a type-I transmembrane protein, can be cleaved in an amyloidogenic and
a non-amyloidogenic pathway. The amyloidogenic pathway is initiated by β-secretase (BACE1) cleavage of APP within its extracellular/luminal domain, generating
soluble β-secreted APP (sAPPβ) and a C-terminal membrane-bound fragment of 99 amino acid residues, βCTF/C99. βCTF/C99 is further cleaved by
γ-secretase, a heterotetrameric protein complex consisting of at least four proteins, PS1 or PS2, Aph1a or Aph1b, nicastrin (Nct) and PEN2. The initial cut by
β-secretase BACE1 generates the N-terminus of Aβ, whereas the final cut of βCTF/C99 by γ-secretase generates the C-terminus of Aβ, thus releasing Aβ peptides.
In the non-amyloidogenic pathway APP is first cleaved by α-secretases, identified as members of the ADAM family (a disintegrin and metalloproteinase)
(ADAM10/ADAM17). Processing by α-secretase generates soluble α-secreted APP (sAPPα) and a C-terminal membrane-bound fragment of 83 amino acid
residues, αCTF/C83, which is further cleaved by the γ-secretase complex to generate the peptide p3. The α-secretase cleaves APP within the Aβ domain,
thus precluding the formation of Aβ peptides. The γ-secretase cleavage of βCTF/C99 and αCTF/C83 liberates the APP intracellular domain (AICD), which
translocates to the nucleus and regulates gene expression [306-310].
BACE1 cleaves APP at the known β-sites Asp1 and Glu11 of the Aβdomain, generating C-terminal membrane-embedded fragments of 99 or 89 amino acids (C99 and C89, respectively) [13,37]. C99 and C89 are subsequently cleaved by γ-secretase within the transmembrane domain, yielding mainly in Aβ1-40/1-42 and Aβ11-40/11-42 [38-41]. Due to the two additional amino acids isoleucine and alanine, Aβ42 peptides are more hydrophobic and aggregate more readily than Aβ40 peptides. Both peptides, full-length Aβand N-terminal truncated Aβpeptides can be found in amyloid plaques of AD brains. Beside the production of Aβ40, which represents with 80-90% of Aβ generated the most abundant species, and Aβ42 peptides, which represent only about 10% of Aβ peptides, additional Aβ isoforms varying in length at the C-terminus of Aβ are released by γ-cleavage [42-44]. The relative unspecificity of γ-secretase might be caused by the unusual intramembrane proteolytic activity of γ-secretase, cleaving APP within the hydrophobic transmembrane domain [45]. The generation of Aβ46 and Aβ49 peptides, also known as ζ-site [46,47] and ε-site cleavage of APP [48,49], suggests as one γ-secretase cleavage model a sequential cleavage of γ-secretase, where cleavage at the ε-site is followed by the ζ-site and finally the γ-cleavage site.
Molecular identification of the γ-secretase has shown that γ-secretase activity resides in a heterotetrameric protein complex. Members of the γ-secretase complex are presenilin1 (PS1) or presenilin 2 (PS2), anterior pharynx defective 1 (APH-1), presenilin enhancer 2 (PEN-2) and nicastrin (NCT) [50,51]. Notably, all components of the γ-secretase complex are, like the substrate APP itself, transmembrane proteins. The assembly of the four components of the γ-secretase leads to the auto- and endoproteolysis of PS into a Nand C-terminal fragment that contribute aspartate residues as active site of the multimeric protease [52-54]. Although all four γ-secretase components are necessary for enzymatic activity, major impact regarding AD pathogenesis belongs to the presenilins. More than 150 autosomal dominant point mutations in the presenilins have been identified, causing familiar early-onset form of AD (FAD) [55,56]. These mutations elevate the generation of Aβ42 peptides [57,58].
Amyloidogenic APP processing by β- and γ-secretase is discussed to be dependent on particular membrane microdomains, called lipid rafts. Lipid rafts are small (10-200nm) dynamic membrane microdomains enriched in cholesterol and sphingolipids.
They are distinct from surrounding non-raft membrane microdomains of unsaturated phospholipids and serve as a platform for diverse cellular processes like signal transduction, cell adhesion and protein trafficking. Lipid rafts are first ensembled in the Golgiapparatus and are highly abundant at the plasmamembrane and the endocytotic pathway. All four members of the heterotetrameric γ-secretase complex as well as BACE1 have been found in lipid raft membrane microdomains [59-61]. Further indications to raftassociated amyloidogenic APP processing are the accumulation of APP C-terminal fragments in lipid raft membrane microdomains in absence of γ-secretase activity [59] and the influence of cholesterol depletion on β- and γ-secretase processing of APP. Cholesterol depletion causes the dissociation of the γ-secretase components from lipid rafts [59] and decreases in general Aβ generation [62-68]. Beside cholesterol, which is important for stabilizing lipid raft membrane microdomains, further lipids have been shown to modulate amyloidogenic APP processing [69-75], indicating that a delicate balance in lipid composition of cellular membranes is crucial for AD pathogenesis. In particular sphingolipids like gangliosides, which are beside cholesterol enriched in raft membrane microdomains, have been implicated in Aβ toxicity. GM1 has been shown to increase Aβ generation [76] and the importance of ganglioside clusters in amyloid fibril formation is of increasing interest. In 1995, Yanagisawa et al. [77] reported that GM1 binds to membrane-bound Aβ (GAβ) which might act as a seed for Aβ aggregation in amyloid plaques [78,79]. Up to date, evidence has increased that binding of gangliosides to Aβ induces a conformational change of Aβ [80-84], which facilitates amyloid fibril formation [85] in the brain. Further studies revealed that the generation of GAβ and the formation of amyloid fibrils preferably occurs in specific membrane microdomains with a lipid raft like composition of sphingomyelin, cholesterol and ganglioside GM1 [86,87]. Notably, the extent of amyloid fibril formation does not only depend on the ganglioside level but is also dependent on cholesterol. Cholesterol has been shown to facilitate the formation of ganglioside clusters important for GAβformation [87] and cholesterol depletion by methyl-β-cyclodextrin, which disrupts lipid raft membrane microdomains, resulted in reduced amyloid fibril formation [88], emphasizing the importance of lipid rafts not only in Aβ generation by β- and γ-secretase but also on the formation of toxic amyloid fibrils.
Similar to the therapeutic inhibition of BACE1 activity, inhibition of the γ-secretase activity to prevent Aβ generation, is not suitable to treat or prevent AD. The γ-secretase complex is beside APP essential for the proteolysis of many type-I transmembrane proteins, for example Notch, ErbB4, CD44, LRP1 and jagged, which exert important functions in normal cellular physiology [89,90]. Therefore a modulation of γ-secretase activity instead of inhibition might be more beneficial to prevent AD or at least to delay the age of disease onset [69,73,75,76].
Non-amyloidogenic APP processing
Beside β-secretase cleavage of APP, APP can be first cleaved by α-secretases in a non-amyloidogenic pathway, thus preventing the generation of Aβ peptides (Figure 1). The α-secretases are members of the ADAM family (a disintegrin and metalloproteinase) and cleave APP within the Aβ domain, generating α-secreted APP (sAPPα) and the C-terminal fragment αCTF, which is cleaved by the γ-secretase complex to generate p3 [38,91-94]. The p3 peptides are not amyloidogenic and sAPPα has neuroprotective and memory enhancing effects [95-97]. α-Secretase processing of APP occurs constitutively (constitutive α-secretase), however additionally α-secretase proteolytic activity can be stimulated by a heterogeneous group of molecules (regulated α-secretase) [98-102]. ADAM proteases are type-I integral membrane proteins of the metzincin family requiring zinc ions for their proteolytic activity [103,104]. So far three members of the ADAM family have been suggested as α-secretases, ADAM9, ADAM10 and ADAM17. ADAM17, also known as TACE (tumor necrosis factor-α converting enzyme), was initially identified as protease responsible for the release of the inflammatory cytokine tumor necrosis factor-α (TNF-α) [105,106]. Several studies implicate ADAM17 in regulated α-cleavage: Fibroblasts of ADAM17/TACE knock-out mice are defective in their protein kinase C stimulated sAPPα secretion [107] and inhibition of ADAM17/TACE in primary neurons leads to the suppression of regulated but not constitutive α-secretase activity [108]. Similarly ADAM9 seems to be involved only in regulated α-cleavage. Phorbol ester treatment promoted sAPPα secretion in COS cells expressing ADAM9 and APP [93], whereas RNAi mediated silnecing of ADAM9 had no effect on constitutive sAPPα generation [109]. Overexpression of ADAM10 in human embryonic kidney cells 293 resulted in the elevation of constitutive and PKC-regulated α-cleavage of APP [92]. However, two recent studies identified ADAM10 to be the constitutive α-secretase in primary neurons [109,110]. RNAi mediated knock-down of ADAM10, but not of ADAM9 or ADAM17, completely suppressed APP α-secretase cleavage in different cell lines and in primary murine neurons [109]. Moreover, ADAM9 or ADAM17 were not able to compensate for this loss of constitutive α-secretase cleavage [109]. Primary neurons of ADAM10 conditional knock-out mice showed reduced α-secretase processing of APP, further demonstrating that ADAM10 represents the most important APP α-secretase in brain [110]. As the non-amyloidogenic processing of APP by α-secretase prevents the formation of amyloidogenic Aβ peptides, an increase in α-secretase activity is an attractive target for the treatment of AD. A further mechanism to modulate Aβ generation might be a shift of amyloidogenic APP processing to non-amyloidogenic processing of APP. The omega-3 fatty acid docosahexaenoic acid (DHA), enriched in marine fish and algae, has been recently described to decrease Aβgeneration in vitro and to decrease amyloid burden in animal models of AD [111-117] via pleiotropic mechanism, including a shift from amyloidogenic to non-amyloidogenic APP processing [73]. Alterations in dietary intake or functional foods might therefore be beneficial for preventing or treating AD [118-120].
Aβ Toxicity
Amyloid plaques consisting of aggregated Aβ peptides are the indisputable characteristic feature of AD, however increasing evidence suggests that small oligomers of Aβ up to 50 Aβ subunits represent the most toxic species of Aβ [121,122]. Up to date several mechanisms of Aβ toxicity have been described, including disruption of calcium homeostasis, cholinergic dysfunction, inflammation, increased oxidative stress and mitochondrial dysfunction (Figure 2) [123-127].
Disruption of calcium homeostasis
Intracellular Ca2+ signalling is fundamental to neuronal function and viability. Because of the ubiquitious involvement of Ca2+ in second-messenger signalling, neurons at rest have strict mechanisms to maintain low Ca2+ concentrations in the cytosol by extruding Ca2+ into the extracellular space and pumping Ca2+ into the endoplasmic reticulum [128]. Upon activation, Ca2+ can flux through voltage-, ligand- or store-operated-channels from the extracellular space into the cytosol or G-protein coupled receptors are stimulated, increasing the generation of inositol-1,4,5-trisphosphate (IP3), which binds to IP3 receptors (IP3R) on the endoplasmic reticulum (ER) to release Ca2+ out of the ER into the cytosol. Elevated cytosolic Ca2+ level trigger neuronal cellular signalling cascades involved in membrane excitability, neurotransmitter release, gene expression, cellular growth, differentiation, free radical species formation and cell death [129].
Aβ induced Ca2+ dyshomeostasis is an important component of AD pathology, altering neurotransmitter receptor properties, disrupting membrane integrity and intitiating apoptotic signalling cascades finally resulting in synaptic degeneration, cell death and devastating memory loss. Aβ has been shown to interact with different Ca2+-permeable channels [130] and to increase Ca2+ entry into the cytosol. Voltage-gated plasmalemmal Ca2+ channels are a major source of cytosolic Ca2+ entry in neurons. Aβ significantly increases Ca2+ influx via activation of voltage-gated Ca2+ channels (VGCCs) of the L-, N- and P-type [130,131], resulting in elevated postsynaptic responses. Beside VGCCs, the Ca2+-permeable receptors of the cholinergic and glutamatergic neurotransmitter system, the AMPA and NMDA receptors, are discussed to be involved in AD pathology. These receptors are expressed in brain regions supporting higher cognitive functions, the hippocampus and neocortex, and these brain areas are primarily affected by the neuronal loss observed in AD [132,133]. Aβ oligomers have been shown to modulate NMDA receptor activity [134-136] and to increase NMDA receptor mediated Ca2+ influx [137,138], causing dynamin-1 degradation and promoting calpain activity [137] important for synaptic vesicle formation and synaptic vesicle function. However, it has also been reported that Aβ oligomers reduce NMDA-receptor expression and therefore Ca2+ influx [139,140].
Cholinergic dysfunction
The malfunction of synaptic integrity is also a consequence of a deregulation in the Ca2+-permeable n-acetylcholine-receptor (nAChR) channels. The most vulnerable neurons in AD are discussed to be those expressing high levels of nAChRs, particularly those containing the α7 subunit [141]. Aβ has been shown to bind to α7- andα4β2-nAChRs in cortical and hippocampal synaptic membrane preparations [142] and to affect nAChR functioning [143]. Furthermore, α7- and α4β2- nACHRs positively correlate with neurons that accumulate Aβ [142] and α7-nAChR colocalizes with amyloid plaques [144]. The dramatic degeneration of cholinergic neurons in AD and the loss of cholinergic neurotransmission contributes to cognitive deterioration in AD [145] and led to the development of pharmaceutical drugs targeting cholinergic molecular components, mainly the breakdown of acetylcholine by acetylcholine-esterase [146].
Figure 2:Molecular mechanisms of AÃ?Â? toxicity. Overview of the molecular mechanisms of AÃ?Â? toxicity leading to reduced neuronal function and viability,
synaptic degeneration, cell death and devastating memory loss.
Beside Aβ induced alterations in Ca2+-permeable plasmalemmal channels or receptors, Aβ influences Ca2+ efflux from the endoplasmic reticulum, the most important intracellular Ca2+ reservoir. Ca2+ can be released out of the ER into the cytosol either by IP3-receptors or ryanodine receptors. Aβ has been shown to stimulate IP3-mediated calcium release from the ER [147] and to increase the expression of G-protein coupled metabotropic glutamate receptor 5, which generates IP3 resulting in Ca2+ release out of the ER [148]. An Aβ induced activation of ryanodine receptors (RyR) has been reported for RyR1 reconstituted in planar lipid bilayers [149] and Aβ42 has been shown to increase the expression of RyR3 [150]. Interestingly, several studies have also linked familial PS mutations, increasing the ratio of Aβ42 to Aβ40 peptides, with elevated Ca2+ release out of the ER [151-154].
Altered Ca2+ homeostasis in AD affected neurons may also be a consequence of the formation of calcium permeable Aβ channels: Aβ peptides incorporate in the lipid bilayer and reorganize to form cation conducting channels in vitro and in intact neurons [155-163]. Recently, the presence of Aβ pores in the cell membrane of post mortem brains of individuals affected by AD but not in healthy individuals have been detected using high resolution transmission electron micoscropy [164]. Furthermore, Diaz et al. [165] reported that blocking Aβ channels by the use of amphiphilic pyridinium salts protect neurons from Aβ neurotoxicity [165].
Decreased membrane integrity
A similar unspecific flux of cations in neuronal plasma membranes is achieved by Aβ induced disruption of membrane lipid integrity. Aβpeptides does not only interact with gangliosides as discussed above, but rather with further membrane lipids like phosphatidylcholine, phosphatidylglycerol and phosphoinositides [166-170], resulting in changed membrane fluidity, discussed to increase membrane permeability to Ca2+, Na+ and K+ ions [80,171]. Interestingly, it has also been reported that lack of PS1/2 results in a decreased membrane fluidity [172]. Independent of the mechanism how Aβ causes Ca2+ dyshomeostasis in AD, the disruption of intracellular Ca2+ signalling finally results in synaptic breakdown, cell death and devastating memory loss.
Increased oxidative stress and mitochondrial dysfunction
Beside influencing neurotransmitter receptor properties and triggering apoptosis [173], destabilization of cytosolic Ca2+ levels increases the formation of reactive oxidative species (ROS), resulting in lipid peroxidation and the oxidation of proteins [174,175]. An increase in cytosolic Ca2+ level can cause excessive Ca2+ flux into mitochondria and increase the production of ROS. In line, Manczak et al. [176] reported mitochondria to be a direct site for Aβ accumulation in AD neurons. Analyzing transgenic mouse models and neuronal cell cultures, Aβ has been found to accumulate in mitochondria, resulting in elevated hydrogen peroxide production and decreased cytochrome-c oxidase activity, leading to mitochondrial oxidative damage, mitochondrial dysfunction and reduced energy metabolism [176], and cell death. Importantly, it is well established that in return oxidative stress itself increases the generation of Aβ [177- 180], emphasizing oxidative stress to be an important factor in the pathogenesis of AD.
Neuroinflammation
Aβ induced activation of immunocompetent cells (microglia and astrocytes) of the brain, leading to neuroinflammation is a further mechanism of Aβ neurotoxicity. Aβ has been shown to activate microglia, however the exact mechanism of Aβ induced microglia activation has not been clearly defined [181]. The involvement of p38 mitogen-activated protein kinase (p38 MAPK), pattern recognition receptors and the toll-like receptors (TLR) in Aβ activated microglia immune response have been discussed [182-184]. Quite recently, Kauppinen et al. [185] reported that the nuclear enzyme poly (ADPribose)- 1 (PARP-1) regulates microglial activation in response to Aβ Injection of Aβ 42 into wildtype mouse brain revealed microglial response, which was blocked in mice lacking PARP- 1 expression. Using microglial cultures they could further show that PARP-1 activity is essential for Aβ-induced NF-κB activation, morphological transformation, nitric oxide release (NO), tumour necrosis factor-α (TNF-α release and neurotoxicity. Treatment of astrocytes with oligomeric Aβ increased the generation of the major pro-inflammatory cytokine interleukin-1 (IL-1), NO and TNF-α in rat astrocyte cultures and lead to increased astrocyte-mediated inflammation [186]. Furthermore oligomeric Aβ correlates with neuronal loss and astrocyte inflammatory response in APP/Tau transgenic mice [187] and astrocytes are important mediators of Aβ- induced neurotoxicity and tau phosphorylation in primary cultures [188]. Similar to the feed-forward cycle that Aβ induces oxidative stress and increased oxidative stress itself elevates Aβ generation, Aβ triggers neuroinflammation, which in turn increases Aβ generation [189-191]. Moreover, oxidative stress leads to the release of proinflammatory cytokine, chemokines and complements, resulting in the activation of microglia and astrocytes [192,193], emphasizing that the described mechanisms of Aβ toxicity gear into each other and that AD is a multifactorial neurodegenerative disease.
Notch and Alzheimer’s Disease
The Notch signalling pathway is also discussed to be implicated in the pathogenesis of AD. Notch receptors are type-I transmembrane proteins, which are processed in a similar way to APP (Figure 3). During its maturation in the secretory pathway, the Notch receptor is constitutively cleaved at the S1 site by a furin-like protease, generating a heterodimeric molecule, which consists of an extracellular and a transmembrane fragment, remaining non-covalently associated [194]. Binding of DSL ligands (Delta/Serrate/LAG-2) of adjacent cells to cell surface localized Notch receptors induces cleavage of Notch at the S2 site. In analogy to α-secretase cleavage of APP, S2 cleavage of Notch is mediated by proteases of the ADAM family [195-198] and is located in the extracellular domain of Notch close to the transmembrane domain. The membrane-embedded remaining Notch fragment NEXT (Notch extracellular truncation) is further processed at the S3 site by the γ-secretase complex [199-201], generating the Notch intracellular fragment (NICD), which translocates to the nucleus, where it participates directly in the transcriptional regulation of target genes by interacting with DNA-binding proteins of the CSL-family (mammalian CBF-1/Drosophila Su(H)/C. elegans LAG-1) [202].
Figure 3:Proteolytic processing of Notch. During its maturation in the secretory pathway, the Notch receptor is constitutively cleaved at the S1 site by a furin-like
protease, generating a non-covalently associated heterodimeric molecule. Upon activation of the Notch signalling pathway by binding of DSL ligands to cell surface
localized Notch receptors, the heterodimeric Notch molecule is cleaved at the S2 site. In analogy to α-secretase cleavage of APP, S2 cleavage of Notch is mediated
by proteases of the ADAM family. The membrane-embedded remaining Notch fragment NEXT (Notch extracellular truncation) is further processed at the S3 site by
the γ-secretase complex, generating the Notch intracellular fragment (NICD), which translocates to the nucleus, where it participates directly in the transcriptional
regulation of target genes involved in the generation, differentiation and survival of neuronal cells.
The Notch signalling pathway is a highly conserved signalling system in multicellular eukaryotes, regulating cell-fate specification and differentiation processes during development, including neuronal development [203-204]. Beside its critical role in neuronal development, the Notch signalling pathway also functions in the adult brain [205], controlling neural stem cell maintenance, morphology, migration, synaptic plasticity and survival of immature and mature neurons and long-term memory related to cognitive function [206-209]. Dysregulated Notch signalling has been implicated in many diseases, e.g. cancer [210,211], CADASIL (cerebral autosomal dominant arteriopathy with subcortical infarcts and leukoencephalopathy) [212], Allagile [213,214], Hajdu-Cheney syndromes [215,216], multiple sclerosis [217] and Down syndrome [218]. Several indices argue for a potential role of the Notch signalling pathway in AD: Notch is expressed at particularly high levels in the hippocampus of adult brains [219], one of the brain regions affected by AD; Notch plays a role in learning and memory [220] and Notch is a substrate of the γ-secretase complex, which is also involved in the generation of amyloid plaques [221]. Notch1 expression has been shown to be elevated more than 2-fold in the hippocampus of sporadic AD patients compared to control human hippocampus [219]. Furthermore, Notch1 colocalizes and interacts with PS1 [219,222] and APP [223]. The APP intracellular domain (AICD), which is like NICD released by γ-secretase proteolytic activity, has been reported to bind to Numb-proteins, known cytosolic Notch inhibitors, and to repress Notch/NICD activity [224]. This study suggests that γ-secretase has opposing effects on Notch signalling, positive by cleavage of Notch at the S3 site and the resulting generation of NICD, which translocates to the nucleus and activates transcription of genes involved in the generation, differentiation and survival of neuronal cells and negative by γ-secretase processing of APP, generating AICD, which inhibits NICD function [224]. The inhibition of Notch function by AICD might accelerate the neurodegenerative process of AD by enhancing synapse loss, neurite dystrophy and neuronal degeneration. Moreover, for FAD linked PS1 mutations inefficient processing of the Notch receptor has been recently reported to be responsible for impaired self-renewal and differentiation of adult brain neural progenitor cells [225], further indicating that alterations in the Notch signalling pathway may contribute to the pathogenesis of AD.
Tau Hallmark
Beside amyloid plaques, neurofibrillary tangles are considered to be a pivotal pathological hallmark of AD. Neurofibrillary tangles (NFTs) consist of insoluble paired helical fragments (PHF) inside neurons composed mainly of hyperphosphorylated tau protein [226- 229]. Tau proteins are mainly expressed in neurons and can be found in axons [230] and the somato-dendritic compartment [231]. They belong to the family of microtubule-associated proteins (MAPs), important for the assembly of tubulin monomers into microtubules, to stabilize the neuronal microtubule network which is essential for maintaining cell shape and axonal transport [232]. Tau is a phosphoprotein and is found in six isoforms in human brain [233]. The microtubule assembly promoting activity of tau, the major MAP of a mature neuron, and the other two neuronal MAPs (MAP1 and MAP2) is regulated by their phosphorylation status [234,235]. In AD tau proteins are hyperphosphorylated and polymerize into paired helical fragments forming intraneuronal neurofibrillary tangles [226]. Tau pathology is not limited to AD but is also present in other dementing disorders like Pick’s disease, progressive supranuclear palsy and corticobasal degeneration [236,237]. However, the morphology of the NFTs and the composition of the tau isoforms differ from those of AD [238].
The degree of tau phosporylation and therefore tau function is regulated by protein kinases and protein phosphatases. So far, 79 potential serine and threonine phosphorylation sites in tau have been described [239-241] and the abnormal hyperphosphorylation as observed in AD is discussed to be caused either by activation of protein kinases or by reduced activity of protein phosphatases.
Protein kinases involved in tau phosphorylation
Glycogen synthase kinase-3β (GSK3β) has been found to be one of the most important kinases responsible for tau hyperphosphorylation [242-244] and promotes tangle-like filament morphology [245]. Overexpression of GSK3β in cultured cells and in transgenic mice [246,247] leads to tau hyperphosphorylation and GSK3β inhibition by lithium chloride reduces tau phosphorylation [248,249]. Beside GSK3β, casein kinase 1 delta, protein kinase A and the calcium and calmodulin dependent protein kinase-II (CaMKII) have been found to phosphorylate phosphorylation sites which are also phosphorylated in tau proteins present in PHFs in AD [250-253]. A further important candidate involved in tau phosphorylation is the cyclin-dependent kinase 5 (CDK5). Besides GSK3β, CDK5 is highly expressed in human brain [254,256] and both kinases have been shown to be associated with all stages of neurofibrillary changes in AD [257,258]. Silencing of CDK5 reduces the phosphorylation of tau in vitro (neuronal cell cultures) and in vivo (wildtype mice) and knock-down of CDK5 strongly decreased the formation of NFTs in hippocampi of triple transgenic AD mice [259]. Overexpression of p25, a cellular activator of CDK5, in primary neurons and in transgenic mice results in tau hyperphosphorylation and tau aggregation and neurofibrillary pathology in mice [260-262]. Mitogen-activated protein (MAP) kinases, including ERK1, ERK2 [263,264], p70S6 kinase [265] and the stress-activated kinases JNK and p38 kinase [266-268], have been shown to be also involved in tau hyperphosphorylation. Recently, the dual-specificity tyrosine-phosphorylated and regulated kinase 1A (Dyrk1A) has been shown to phosphorylate tau at several phosphorylation sites [269,270]. Notably, tau phosphorylation by Dyrk1A primes tau for further phosphorylation by GSK3β [269].
Protein phosphatases involved in tau dephosphorylation
Several protein phosphatases (PPs), including PP1, PP2A, PP2B and PP5 have been proposed to be involved in tau dephosphorylation in vitro and in vivo [271]. However, PP-2A and PP-1 contribute more than 90% of the serine/threonine protein phosphatase activity in mammalian [272]. PP2A is discussed to be the main enzyme that prevents hyperphosphorylation of tau. Decreased PP2A mRNA expression and reduced PP2A activity have been detected in brains of AD affected individuals [273,274]. PP2A also regulates the activities of several tau kinases, including ERK1, ERK2, p70S6 kinase, CaM Kinase II and PKA. Reduced PP2A activity as observed in AD brain promotes tau phosphorylation by these kinases, emphasizing the role of PP2A in AD by directly decreasing tau dephosphorylation and indirectly by promoting tau phosphorylation. Beside PP2A, which has been show to be reduced in AD brain, the activity of protein phosphatase PP1 has been also shown to be reduced in AD brain [271,274,275], emphasizing the impact of PP2A and PP1 in neurofibrillary changes in AD.
Quite recently, a further protein, early growth response 1 (Egr-1), was described to be involved in tau pathology. Egr-1 is a transcription factor significantly upregulated in AD brain [276,277]. Lu et al. [278] recently identified the pathological significance of this upregulation. Overexpression of Egr-1 controls both, phosphorylation and dephosphorylation of tau, by activating CDK5 and inactivating PP1, leading to tau hyperphosphorylation and destabilized microtubules.
Tau toxicity
Beside polymerization of hyperphosphorylated tau in neurofibrillary tangles, hyperphosphorylated tau might cause conformational changes [279,280] and cleavage of tau by caspases [281,282]. Truncated tau is discussed to be a more favorable substrate for abnormal hyperphosphorylation as truncated tau promotes tau hyperphosphorylation and neurofibrillary degeneration in vivo in transgenic rats [283]. Furthermore, hyperphosphorylated tau is resistant to degradation by calpains [284,285], calcium activated neutral proteases and the proteasome [286], indicating that increased tau level observed in AD brain [287] are caused by a decrease in its turnover.
In the axons, non-fibrillized hyperphosphorylated tau is discussed to disrupt microtubules by sequestering normal tau and the two other neuronal MAPs, MAP1 and MAP2, whereas its aggregation as neurofibrillary tangles probably impairs axoplasmic flow and leads to slow progressive retrograde degeneration and loss of connectivity of the affected neurons [288]. In the somato-dendritic compartment hyperphosphorylated tau has been found to influence intracellular compartments by inducing fragmentation of the Golgi apparatus and by reducing mitochondria and rough endoplasmic reticulum [289,290]. The accumulation of hyperphosphorylated tau in the ER might cause neurodegeneration due to prolonged ER stress [288,291].
Linking Tau and Amyloid Pathology
Although Aβ and tau exert their neurotoxic effects through separate mechnisms, several in vitro and in vivo studies suggest a link between Aβ and tau pathology. As described tau pathology is caused by the hyperphosphorylation of tau, which is regulated by kinases and phosphatases. Aβ has been shown to activate GSK3β, one of the main kinases involved in tau phosphorylation, leading to aggravated tau pathology in the hippocampus and cortex of a transgenic mouse model, expressing mutant APP and mutant tau [292]. Furthermore, mutations in PS increased GSK3 activity and tau hyperphosphorylation in cell culture experiments [293]. More recent studies revealed that double transgenic mice, expressing mutant tau and mutant APP showed enhanced neurofibrillary tangle pathology in the limbic system and olfactory cortex compared to mice expressing solely mutant tau [294] and that intracranial injection of synthetic Aβ42 accelerates neurofibrillary tangle formation in mutant tau transgenic mice [295]. In return, immunization against Aβ revealed reduced levels of hyperphosphorylated tau in triple transgenic mice [296]. However, the causal link between aberrant APP processing and tau pathology in AD remains controversial as double transgenic mice expressing mutant APP and mutant tau show enhanced amyloid deposition compared to single transgenic mice [297]. Furthermore, knock-out of tau protects neurons from Aβ-induced cell death [298-300]. One of these studies implicated NMDA-receptors to be involved in reduced Aβ neurotoxicity [299]. As described above, Aβ is discussed to exert its toxic effect via alteration in NMDA-receptor function. Ittner et al. [299] recently described that tau is responsible for postsynaptic targeting of the src-kinase Fyn, which phosphorylates NMDA-receptors. Reduced dendritic localization of Fyn in animals lacking tau resulted in reduced interaction of NMDRs with PSD95 and as a consequence reduced Aβ-mediated excitotoxicity [299], suggesting that tau-dependent dendritic signalling is crucial for mediating Aβ neurotoxicity.
Further research regarding the cellular function of tau proteins and especially APP [66,301-305] is necessary to understand the link between tau and amyloid pathology and to further identify the mechanism involved in the development of AD.
References
- Ferri CP, Prince M, Brayne C, Brodaty H, Fratiglioni L, et al. (2005) Global prevalence of dementia: a Delphi consensus study. Lancet 366: 2112-2117.
- von Strauss E, Viitanen M, De Ronchi D, Winblad B, Fratiglioni L (1999) Aging and the occurrence of dementia: findings from a population-based cohort with a large sample of nonagenarians. Arch Neurol 56: 587-592.
- Selkoe DJ (2004) Cell biology of protein misfolding: the examples of Alzheimer's and Parkinson's diseases. Nat Cell Biol 6: 1054-1061.
- Glenner GG, Wong CW (1984) Alzheimer's disease: initial report of the purification and characterization of a novel cerebrovascular amyloid protein. Biochem Biophys Res Commun 120: 885-890.
- Dyrks T, Weidemann A, Multhaup G, Salbaum JM, Lemaire HG, et al. (1988) Identification, transmembrane orientation and biogenesis of the amyloid A4 precursor of Alzheimer's disease. EMBO J 7: 949-957.
- Wasco W, Bupp K, Magendantz M, Gusella JF, Tanzi RE, et al. (1992) Identification of a mouse brain cDNA that encodes a protein related to the Alzheimer disease-associated amyloid beta protein precursor. Proc Natl Acad Sci U S A 89: 10758-10762.
- Wasco W, Gurubhagavatula S, Paradis MD, Romano DM, Sisodia SS, et al. (1993) Isolation and characterization of APLP2 encoding a homologue of the Alzheimer's associated amyloid beta protein precursor. Nat Genet 5: 95-100.
- Jacobsen KT, Iverfeldt K (2009) Amyloid precursor protein and its homologues: a family of proteolysis-dependent receptors. Cell Mol Life Sci 66: 2299-2318.
- Buée- L, Bussière T, Buée--Scherrer V, Delacourte A, Hof PR (2000) Tau protein isoforms, phosphorylation and role in neurodegenerative disorders. Brain Res Brain Res Rev 33: 95-130.
- Binder LI, Guillozet-Bongaarts AL, Garcia-Sierra F, Berry RW (2005) Tau, tangles, and Alzheimer's disease. Biochim Biophys Acta 1739: 216-223.
- Mohamed A, Cortez L, de Chaves EP (2011) Aggregation state and neurotoxic properties of alzheimer beta-amyloid peptide. Curr Protein Pept Sci 12: 235-257.
- Sinha S, Anderson JP, Barbour R, Basi GS, Caccavello R, et al. (1999) Purification and cloning of amyloid precursor protein beta-secretase from human brain. Nature 402: 537-540.
- Vassar R, Bennett BD, Babu-Khan S, Kahn S, Mendiaz EA, et al. (1999) Beta-secretase cleavage of Alzheimer's amyloid precursor protein by the transmembrane aspartic protease BACE. Science 286: 735-741.
- Yan R, Bienkowski MJ, Shuck ME, Miao H, Tory MC, et al. (1999) Membrane-anchored aspartyl protease with Alzheimer's disease beta-secretase activity. Nature 402: 533-537.
- Cai H, Wang Y, McCarthy D, Wen H, Borchelt DR, et al. (2001) BACE1 is the major beta-secretase for generation of Abeta peptides by neurons. Nat Neurosci 4: 233-234.
- Luo Y, Bolon B, Kahn S, Bennett BD, Babu-Khan S, et al. (2001) Mice deficient in BACE1, the Alzheimer's beta-secretase, have normal phenotype and abolished beta-amyloid generation. Nat Neurosci 4: 231-232.
- Roberds SL, Anderson J, Basi G, Bienkowski MJ, Branstetter DG, et al. (2001) BACE knockout mice are healthy despite lacking the primary beta-secretase activity in brain: implications for Alzheimer's disease therapeutics. Hum Mol Genet 10: 1317-1324.
- Dominguez D, Tournoy J, Hartmann D, Huth T, Cryns K, et al. (2005) Phenotypic and biochemical analyses of BACE1- and BACE2-deficient mice. J Biol Chem 280: 30797-30806.
- Kim DY, Ingano LA, Carey BW, Pettingell WH, Kovacs DM (2005) Presenilin/gamma-secretase-mediated cleavage of the voltage-gated sodium channel beta2-subunit regulates cell adhesion and migration. J Biol Chem 280: 23251-23261.
- Wong HK, Sakurai T, Oyama F, Kaneko K, Wada K, et al. (2005) beta Subunits of voltage-gated sodium channels are novel substrates of beta-site amyloid precursor protein-cleaving enzyme (BACE1) and gamma-secretase. J Biol Chem 280: 23009-23017.
- Hu X, Hicks CW, He W, Wong P, Macklin WB, et al. (2006) Bace1 modulates myelination in the central and peripheral nervous system. Nat Neurosci 9: 1520-1525.
- Willem M, Garratt AN, Novak B, Citron M, Kaufmann S, et al. (2006) Control of peripheral nerve myelination by the beta-secretase BACE1. Science 314: 664-666.
- Falls DL (2003) Neuregulins: functions, forms, and signaling strategies. Exp Cell Res 284: 14-30.
- Michailov GV, Sereda MW, Brinkmann BG, Fischer TM, Haug B, et al. (2004) Axonal neuregulin-1 regulates myelin sheath thickness. Science 304: 700-703.
- Kim DY, Gersbacher MT, Inquimbert P, Kovacs DM (2011) Reduced sodium channel Na(v)1.1 levels in BACE1-null mice. J Biol Chem 286: 8106-8116.
- Nikolaev A, McLaughlin T, O'Leary DD, Tessier-Lavigne M (2009) APP binds DR6 to trigger axon pruning and neuron death via distinct caspases. Nature 457: 981-989.
- Bennett BD, Babu-Khan S, Loeloff R, Louis JC, Curran E, et al. (2000) Expression analysis of BACE2 in brain and peripheral tissues. J Biol Chem 275: 20647-20651.
- Farzan M, Schnitzler CE, Vasilieva N, Leung D, Choe H (2000) BACE2, a beta -secretase homolog, cleaves at the beta site and within the amyloid-beta region of the amyloid-beta precursor protein. Proc Natl Acad Sci U S A 97: 9712-9717.
- Walter J, Fluhrer R, Hartung B, Willem M, Kaether C, et al. (2001) Phosphorylation regulates intracellular trafficking of beta-secretase. J Biol Chem 276: 14634-14641.
- Vassar R, Kovacs DM, Yan R, Wong PC (2009) The beta-secretase enzyme BACE in health and Alzheimer's disease: regulation, cell biology, function, and therapeutic potential. J Neurosci 29: 12787-12794.
- Huse JT, Pijak DS, Leslie GJ, Lee VM, Doms RW (2000) Maturation and endosomal targeting of beta-site amyloid precursor protein-cleaving enzyme. The Alzheimer's disease beta-secretase. J Biol Chem 275: 33729-33737.
- Huse JT, Liu K, Pijak DS, Carlin D, Lee VM, et al. (2002) Beta-secretase processing in the trans-Golgi network preferentially generates truncated amyloid species that accumulate in Alzheimer's disease brain. J Biol Chem 277: 16278-16284.
- Hook V, Toneff T, Bogyo M, Greenbaum D, Medzihradszky KF, et al. (2005) Inhibition of cathepsin B reduces beta-amyloid production in regulated secretory vesicles of neuronal chromaffin cells: evidence for cathepsin B as a candidate beta-secretase of Alzheimer's disease. Biol Chem 386: 931-940.
- Hook VY, Kindy M, Reinheckel T, Peters C, Hook G (2009) Genetic cathepsin B deficiency reduces beta-amyloid in transgenic mice expressing human wild-type amyloid precursor protein. Biochem Biophys Res Commun 386: 284-288.
- Mueller-Steiner S, Zhou Y, Arai H, Roberson ED, Sun B, et al. (2006) Antiamyloidogenic and neuroprotective functions of cathepsin B: implications for Alzheimer's disease. Neuron 51: 703-714.
- Sun B, Zhou Y, Halabisky B, Lo I, Cho SH, et al. (2008) Cystatin C-cathepsin B axis regulates amyloid beta levels and associated neuronal deficits in an animal model of Alzheimer's disease. Neuron 60: 247-257.
- Gouras GK, Xu H, Jovanovic JN, Buxbaum JD, Wang R, et al. (1998) Generation and regulation of beta-amyloid peptide variants by neurons. J Neurochem 71: 1920-1925.
- Haass C, Schlossmacher MG, Hung AY, Vigo-Pelfrey C, Mellon A, et al. (1992) Amyloid beta-peptide is produced by cultured cells during normal metabolism. Nature 359: 322-325.
- Shoji M, Golde TE, Ghiso J, Cheung TT, Estus S, et al. (1992) Production of the Alzheimer amyloid beta protein by normal proteolytic processing. Science 258: 126-129.
- Scheuner D, Eckman C, Jensen M, Song X, Citron M, et al. (1996) Secreted amyloid beta-protein similar to that in the senile plaques of Alzheimer's disease is increased in vivo by the presenilin 1 and 2 and APP mutations linked to familial Alzheimer's disease. Nat Med 2: 864-870.
- Fukuyama R, Mizuno T, Mori S, Nakajima K, Fushiki S, et al. (2000) Age-dependent change in the levels of Abeta40 and Abeta42 in cerebrospinal fluid from control subjects, and a decrease in the ratio of Abeta42 to Abeta40 level in cerebrospinal fluid from Alzheimer's disease patients. Eur Neurol 43: 155-160.
- Wang R, Sweeney D, Gandy SE, Sisodia SS (1996) The profile of soluble amyloid beta protein in cultured cell media. Detection and quantification of amyloid beta protein and variants by immunoprecipitation-mass spectrometry. J Biol Chem 271: 31894-31902.
- Grimm HS, Beher D, Lichtenthaler SF, Shearman MS, Beyreuther K, et al. (2003) gamma-Secretase cleavage site specificity differs for intracellular and secretory amyloid beta. J Biol Chem 278: 13077-13085.
- Schieb H, Kratzin H, Jahn O, Möbius W, Rabe S, et al. (2011) Beta-amyloid peptide variants in brains and cerebrospinal fluid from amyloid precursor protein (APP) transgenic mice: comparison with human Alzheimer amyloid. J Biol Chem 286: 33747-33758.
- Grziwa B, Grimm MO, Masters CL, Beyreuther K, Hartmann T, et al. (2003) The transmembrane domain of the amyloid precursor protein in microsomal membranes is on both sides shorter than predicted. J Biol Chem 278: 6803-6808.
- Zhao G, Mao G, Tan J, Dong Y, Cui MZ, et al. (2004) Identification of a new presenilin-dependent zeta-cleavage site within the transmembrane domain of amyloid precursor protein. J Biol Chem 279: 50647-50650.
- Zhao G, Tan J, Mao G, Cui MZ, Xu X (2007) The same gamma-secretase accounts for the multiple intramembrane cleavages of APP. J Neurochem 100: 1234-1246.
- Sastre M, Steiner H, Fuchs K, Capell A, Multhaup G, et al. (2001) Presenilin-dependent gamma-secretase processing of beta-amyloid precursor protein at a site corresponding to the S3 cleavage of Notch. EMBO Rep 2: 835-841.
- Weidemann A, Eggert S, Reinhard FB, Vogel M, Paliga K, et al. (2002) A novel epsilon-cleavage within the transmembrane domain of the Alzheimer amyloid precursor protein demonstrates homology with Notch processing. Biochemistry 41: 2825-2835.
- Kimberly WT, LaVoie MJ, Ostaszewski BL, Ye W, Wolfe MS, et al. (2003) Gamma-secretase is a membrane protein complex comprised of presenilin, nicastrin, Aph-1, and Pen-2. Proc Natl Acad Sci U S A 100: 6382-6387.
- Takasugi N, Tomita T, Hayashi I, Tsuruoka M, Niimura M, et al. (2003) The role of presenilin cofactors in the gamma-secretase complex. Nature 422: 438-441.
- Borchelt DR, Thinakaran G, Eckman CB, Lee MK, Davenport F, et al. (1996) Familial Alzheimer's disease-linked presenilin 1 variants elevate Abeta1-42/1-40 ratio in vitro and in vivo. Neuron 17: 1005-1013.
- Wolfe MS, Xia W, Ostaszewski BL, Diehl TS, Kimberly WT, et al. (1999) Two transmembrane aspartates in presenilin-1 required for presenilin endoproteolysis and gamma-secretase activity. Nature 398: 513-517.
- Ahn K, Shelton CC, Tian Y, Zhang X, Gilchrist ML, et al. (2010) Activation and intrinsic gamma-secretase activity of presenilin 1. Proc Natl Acad Sci U S A 107: 21435-21440.
- St George-Hyslop PH, Petit A (2005) Molecular biology and genetics of Alzheimer's disease. C R Biol 328: 119-130.
- Duering M, Grimm MO, Grimm HS, Schröder J, Hartmann T (2005) Mean age of onset in familial Alzheimer's disease is determined by amyloid beta 42. Neurobiol Aging 26: 785-788.
- Duff K, Eckman C, Zehr C, Yu X, Prada CM, et al. (1996) Increased amyloid-beta42(43) in brains of mice expressing mutant presenilin 1. Nature 383: 710-713.
- Kowalska A (2004) Genetic aspects of amyloid beta-protein fibrillogenesis in Alzheimer's disease. Folia Neuropathol 42: 235-237.
- Vetrivel KS, Cheng H, Lin W, Sakurai T, Li T, et al. (2004) Association of gamma-secretase with lipid rafts in post-Golgi and endosome membranes. J Biol Chem 279: 44945-44954.
- Riddell DR, Christie G, Hussain I, Dingwall C (2001) Compartmentalization of beta-secretase (Asp2) into low-buoyant density, noncaveolar lipid rafts. Curr Biol 11: 1288-1293.
- Vetrivel KS, Cheng H, Kim SH, Chen Y, Barnes NY, et al. (2005) Spatial segregation of gamma-secretase and substrates in distinct membrane domains. J Biol Chem 280: 25892-25900.
- Refolo LM, Pappolla MA, LaFrancois J, Malester B, Schmidt SD, et al. (2001) A cholesterol-lowering drug reduces beta-amyloid pathology in a transgenic mouse model of Alzheimer's disease. Neurobiol Dis 8: 890-899.
- Buxbaum JD, Geoghagen NS, Friedhoff LT (2001) Cholesterol depletion with physiological concentrations of a statin decreases the formation of the Alzheimer amyloid Abeta peptide. J Alzheimers Dis 3: 221-229.
- Wahrle S, Das P, Nyborg AC, McLendon C, Shoji M, et al. (2002) Cholesterol-dependent gamma-secretase activity in buoyant cholesterol-rich membrane microdomains. Neurobiol Dis 9: 11-23.
- Fassbender K, Simons M, Bergmann C, Stroick M, Lutjohann D, et al. (2001) Simvastatin strongly reduces levels of Alzheimer's disease beta -amyloid peptides Abeta 42 and Abeta 40 in vitro and in vivo. Proc Natl Acad Sci U S A 98: 5856-5861.
- Grösgen S, Grimm MO, Friess P, Hartmann T (2010) Role of amyloid beta in lipid homeostasis. Biochim Biophys Acta 1801: 966-974.
- Hartmann T, Kuchenbecker J, Grimm MO (2007) Alzheimer's disease: the lipid connection. J Neurochem 1: 159-170.
- Grimm MO, Grimm HS, Tomic I, Beyreuther K, Hartmann T, et al. (2008) Independent inhibition of Alzheimer disease beta- and gamma-secretase cleavage by lowered cholesterol levels. J Biol Chem 283: 11302-11311.
- Grimm MO, Grimm HS, Pätzold AJ, Zinser EG, Halonen R, et al. (2005) Regulation of cholesterol and sphingomyelin metabolism by amyloid-beta and presenilin. Nat Cell Biol 7: 1118-1123.
- Osenkowski P, Ye W, Wang R, Wolfe MS, Selkoe DJ (2008) Direct and potent regulation of gamma-secretase by its lipid microenvironment. J Biol Chem 283: 22529-22540.
- Rothhaar TL, Grösgen S, Haupenthal VJ, Burg VK, Hundsdörfer B, et al. (2011) Plasmalogens inhibit APP processing by directly affecting gamma-secretase activity in Alzheimer’s disease. The Scientific Word Journal in press.
- Grimm MO, Tomic I, Hartmann T (2002) Potential external source of A beta in biological samples. Nat Cell Biol 4: E164-165.
- Grimm MO, Kuchenbecker J, Grosgen S, Burg VK, Hundsdorfer B, et al. (2011) Docosahexaenoic acid reduces amyloid beta production via multiple pleiotropic mechanisms. J Biol Chem 286: 14028-14039.
- Wolozin B (2004) Cholesterol and the biology of Alzheimer's disease. Neuron 41: 7-10.
- Grimm MO, Rothhaar TL, Grosgen S, Burg VK, Hundsdorfer B, et al. (2011) Trans fatty acids enhance amyloidogenic processing of the Alzheimer Amyloid Precursor Protein (APP). J Nut Biochem.
- Zha Q, Ruan Y, Hartmann T, Beyreuther K, Zhang D (2004) GM1 ganglioside regulates the proteolysis of amyloid precursor protein. Mol Psychiatry 9: 946-952.
- Yanagisawa K, Odaka A, Suzuki N, Ihara Y (1995) GM1 ganglioside-bound amyloid beta-protein (A beta): a possible form of preamyloid in Alzheimer's disease. Nature medicine 1: 1062-1066.
- Wakabayashi M, Okada T, Kozutsumi Y, Matsuzaki K (2005) GM1 ganglioside-mediated accumulation of amyloid beta-protein on cell membranes. Biochem Biophys Res Commun 328: 1019-1023.
- Okada T, Wakabayashi M, Ikeda K, Matsuzaki K (2007) Formation of toxic fibrils of Alzheimer's amyloid beta-protein-(1-40) by monosialoganglioside GM1, a neuronal membrane component. J Mol Biol 371: 481-489.
- McLaurin J, Chakrabartty A (1996) Membrane disruption by Alzheimer beta-amyloid peptides mediated through specific binding to either phospholipids or gangliosides. Implications for neurotoxicity. J Biol Chem 271: 26482-26489.
- Choo-Smith LP, Surewicz WK (1997) The interaction between Alzheimer amyloid beta(1-40) peptide and ganglioside GM1-containing membranes. FEBS Lett 402: 95-98.
- Matsuzaki K, Horikiri C (1999) Interactions of amyloid beta-peptide (1-40) with ganglioside-containing membranes. Biochemistry 38: 4137-4142.
- Williamson MP, Suzuki Y, Bourne NT, Asakura T (2006) Binding of amyloid beta-peptide to ganglioside micelles is dependent on histidine-13. Biochem J 397: 483-490.
- Utsumi M, Yamaguchi Y, Sasakawa H, Yamamoto N, Yanagisawa K, et al. (2008) Up-and-down topological mode of amyloid beta-peptide lying on hydrophilic/hydrophobic interface of ganglioside clusters. Glycoconj J 26: 999-1006.
- Choo-Smith LP, Garzon-Rodriguez W, Glabe CG, Surewicz WK (1997) Acceleration of amyloid fibril formation by specific binding of Abeta-(1-40) peptide to ganglioside-containing membrane vesicles. J Biol Chem 272: 22987-22990.
- Kakio A, Nishimoto S, Yanagisawa K, Kozutsumi Y, Matsuzaki K (2002) Interactions of amyloid beta-protein with various gangliosides in raft-like membranes: importance of GM1 ganglioside-bound form as an endogenous seed for Alzheimer amyloid. Biochemistry 41: 7385-7390.
- Kakio A, Nishimoto SI, Yanagisawa K, Kozutsumi Y, Matsuzaki K (2001) Cholesterol-dependent formation of GM1 ganglioside-bound amyloid beta-protein, an endogenous seed for Alzheimer amyloid. J Biol Chem 276: 24985-24990.
- Yamamoto N, Matsubara T, Sato T, Yanagisawa K (2008) Age-dependent high-density clustering of GM1 ganglioside at presynaptic neuritic terminals promotes amyloid beta-protein fibrillogenesis. Biochim Biophys Acta 1778: 2717-2726.
- Parks AL, Curtis D (2007) Presenilin diversifies its portfolio. Trends Genet 23: 140-150.
- Woo HN, Park JS, Gwon AR, Arumugam TV, Jo DG (2009) Alzheimer's disease and Notch signaling. Biochem Biophys Res Commun 390: 1093-1097.
- Haass C, Hung AY, Schlossmacher MG, Teplow DB, Selkoe DJ (1993) beta-Amyloid peptide and a 3-kDa fragment are derived by distinct cellular mechanisms. J Biol Chem 268: 3021-3024.
- Lammich S, Kojro E, Postina R, Gilbert S, Pfeiffer R, et al. 1999. Constitutive and regulated alpha-secretase cleavage of Alzheimer's amyloid precursor protein by a disintegrin metalloprotease. Proc Natl Acad Sci U S A 96: 3922-3927.
- Koike H, Tomioka S, Sorimachi H, Saido TC, Maruyama K, et al. (1999) Membrane-anchored metalloprotease MDC9 has an alpha-secretase activity responsible for processing the amyloid precursor protein. Biochem J 2: 371-375.
- Allinson TM, Parkin ET, Turner AJ, Hooper NM (2003) ADAMs family members as amyloid precursor protein alpha-secretases. J Neurosci Res 74: 342-352.
- Dulin F, Leveille F, Ortega JB, Mornon JP, Buisson A (2008) P3 peptide, a truncated form of A beta devoid of synaptotoxic effect, does not assemble into soluble oligomers. FEBS Lett 582: 1865-1870.
- Furukawa K, Sopher BL, Rydel RE, Begley JG, Pham DG, et al. (1996) Increased activity-regulating and neuroprotective efficacy of alpha-secretase-derived secreted amyloid precursor protein conferred by a C-terminal heparin-binding domain. J Neurochem 67: 1882-1896.
- Meziane H, Dodart JC, Mathis C, Little S, Clemens J, et al. (1998) Memory-enhancing effects of secreted forms of the beta-amyloid precursor protein in normal and amnestic mice. Proc Natl Acad Sci U S A 95: 12683-12688.
- Nitsch RM, Slack BE, Wurtman RJ, Growdon JH (1992) Release of Alzheimer amyloid precursor derivatives stimulated by activation of muscarinic acetylcholine receptors. Science 258: 304-307.
- Buxbaum JD, Koo EH, Greengard P (1993) Protein phosphorylation inhibits production of Alzheimer amyloid beta/A4 peptide. Proc Natl Acad Sci U S A 90: 9195-9198.
- LeBlanc AC, Koutroumanis M, Goodyer CG (1998) Protein kinase C activation increases release of secreted amyloid precursor protein without decreasing Abeta production in human primary neuron cultures. J Neurosci 18: 2907-2913.
- Hwang EM, Kim SK, Sohn JH, Lee JY, Kim Y, et al. (2006) Furin is an endogenous regulator of alpha-secretase associated APP processing. Biochem Biophys Res Commun 349: 654-659.
- Bandyopadhyay S, Goldstein LE, Lahiri DK, Rogers JT (2007) Role of the APP non-amyloidogenic signaling pathway and targeting alpha-secretase as an alternative drug target for treatment of Alzheimer's disease. Curr Med Chem 14: 2848-2864.
- Roberts SB, Ripellino JA, Ingalls KM, Robakis NK, Felsenstein KM (1994) Non-amyloidogenic cleavage of the beta-amyloid precursor protein by an integral membrane metalloendopeptidase. J Biol Chem 269: 3111-3116.
- Edwards DR, Handsley MM, Pennington CJ (2008) The ADAM metalloproteinases. Mol Aspects Med 29: 258-289.
- Black RA, Rauch CT, Kozlosky CJ, Peschon JJ, Slack JL, et al. (1997) A metalloproteinase disintegrin that releases tumour-necrosis factor-alpha from cells. Nature 385: 729-733.
- Moss ML, Jin SL, Milla ME, Bickett DM, Burkhart W, et al. (1997) Cloning of a disintegrin metalloproteinase that processes precursor tumour-necrosis factor-alpha. Nature 385: 733-736.
- Buxbaum JD, Liu KN, Luo Y, Slack JL, Stocking KL, et al. (1998) Evidence that tumor necrosis factor alpha converting enzyme is involved in regulated alpha-secretase cleavage of the Alzheimer amyloid protein precursor. J Biol Chem 273: 27765-27767.
- Blacker M, Noe MC, Carty TJ, Goodyer CG, LeBlanc AC (2002) Effect of tumor necrosis factor-alpha converting enzyme (TACE) and metalloprotease inhibitor on amyloid precursor protein metabolism in human neurons. J Neurochem 83: 1349-1357.
- Kuhn PH, Wang H, Dislich B, Colombo A, Zeitschel U, et al. (2010) ADAM10 is the physiologically relevant, constitutive alpha-secretase of the amyloid precursor protein in primary neurons. EMBO J 29: 3020-3032.
- Jorissen E, Prox J, Bernreuther C, Weber S, Schwanbeck R, et al. (2010) The disintegrin/metalloproteinase ADAM10 is essential for the establishment of the brain cortex. J Neurosci 30: 4833-4844.
- Lim GP, Calon F, Morihara T, Yang F, Teter B, et al. (2005) A diet enriched with the omega-3 fatty acid docosahexaenoic acid reduces amyloid burden in an aged Alzheimer mouse model. J Neurosci 25: 3032-3040.
- Lukiw WJ, Cui JG, Marcheselli VL, Bodker M, Botkjaer A, et al. (2005) A role for docosahexaenoic acid-derived neuroprotectin D1 in neural cell survival and Alzheimer disease. J Clin Invest 115: 2774-2783.
- Perez SE, Berg BM, Moore KA, He B, Counts SE, et al. (2010) DHA diet reduces AD pathology in young APPswe/PS1 Delta E9 transgenic mice: possible gender effects. J Neurosci Res 88: 1026-1040.
- Oksman M, Iivonen H, Hogyes E, Amtul Z, Penke B, et al. (2006) Impact of different saturated fatty acid, polyunsaturated fatty acid and cholesterol containing diets on beta-amyloid accumulation in APP/PS1 transgenic mice. Neurobiol Dis 23: 563-572.
- Calon F, Lim GP, Yang F, Morihara T, Teter B, et al. (2004) Docosahexaenoic acid protects from dendritic pathology in an Alzheimer's disease mouse model. Neuron 43: 633-645.
- Hooijmans CR, Rutters F, Dederen PJ, Gambarota G, Veltien A, et al. (2007) Changes in cerebral blood volume and amyloid pathology in aged Alzheimer APP/PS1 mice on a docosahexaenoic acid (DHA) diet or cholesterol enriched Typical Western Diet (TWD). Neurobiol Dis 28: 16-29.
- Cole GM, Ma QL, Frautschy SA (2009) Omega-3 fatty acids and dementia. Prostaglandins Leukot Essent Fatty Acids 81: 213-221.
- Kamphuis PJ, Scheltens P (2010) Can nutrients prevent or delay onset of Alzheimer's disease? J Alzheimers Dis 20: 765-775.
- Scheltens P, Kamphuis PJ, Verhey FR, Olde Rikkert MG, Wurtman RJ, et al. (2010) Efficacy of a medical food in mild Alzheimer's disease: A randomized, controlled trial. Alzheimers Dement 6: 1-10 e11.
- Grimm MO, Grosgen S, Riemenschneider M, Tanila H, Grimm HS, et al. (2011) From brain to food: analysis of phosphatidylcholins, lyso-phosphatidylcholins and phosphatidylcholin-plasmalogens derivates in Alzheimer's disease human post mortem brains and mice model via mass spectrometry. J Chromatogr A 1218: 7713-7722.
- Kayed R, Head E, Thompson JL, McIntire TM, Milton SC, et al. (2003) Common structure of soluble amyloid oligomers implies common mechanism of pathogenesis. Science 300: 486-489.
- Shankar GM, Li S, Mehta TH, Garcia-Munoz A, Shepardson NE, et al. (2008) Amyloid-beta protein dimers isolated directly from Alzheimer's brains impair synaptic plasticity and memory. Nature medicine 14: 837-842.
- Demuro A, Parker I, Stutzmann GE (2010). Calcium signaling and amyloid toxicity in Alzheimer disease. J Biol Chem 285: 12463-12468.
- Jomova K, Vondrakova D, Lawson M, Valko M (2010) Metals, oxidative stress and neurodegenerative disorders. Mol Cell Biochem 345: 91-104.
- Mandrekar-Colucci S, Landreth GE (2010) Microglia and inflammation in Alzheimer's disease. CNS Neurol Disord Drug Targets 9: 156-167.
- Fedrizzi L, Carafoli E (2011) Ca2+ dysfunction in neurodegenerative disorders: Alzheimer's disease. Biofactors 37: 189-196.
- Gotz J, Eckert A, Matamales M, Ittner LM, Liu X (2011) Modes of Abeta toxicity in Alzheimer's disease. Cell Mol Life Sci 68: 3359-3375.
- Verkhratsky A, Mattson MP, Toescu EC (2004) Aging in the mind. Trends Neurosci 27: 577-578.
- Berridge MJ (1998) Neuronal calcium signaling. Neuron 21: 13-26.
- Rovira C, Arbez N, Mariani J (2002) Abeta(25-35) and Abeta(1-40) act on different calcium channels in CA1 hippocampal neurons. Biochem Biophys Res Commun 296: 1317-1321.
- MacManus A, Ramsden M, Murray M, Henderson Z, Pearson HA, et al. (2000) Enhancement of (45) Ca(2+) influx and voltage-dependent Ca(2+) channel activity by beta-amyloid-(1-40) in rat cortical synaptosomes and cultured cortical neurons. Modulation by the proinflammatory cytokine interleukin-1beta. J Biol Chem 275: 4713-4718.
- Terry AV Jr, Buccafusco JJ (2003) The cholinergic hypothesis of age and Alzheimer's disease-related cognitive deficits: recent challenges and their implications for novel drug development. J Pharmacol Exp Ther 306: 821-827.
- Kadir a, Almkvist O, Wall a, Langstrom B, Nordberg A (2006) PET imaging of cortical 11C-nicotine binding correlates with the cognitive function of attention in Alzheimer's disease. Psychopharmacology (Berl) 188: 509-520.
- Ye C, Walsh DM, Selkoe DJ, Hartley DM (2004) Amyloid beta-protein induced electrophysiological changes are dependent on aggregation state: N-methyl-D-aspartate (NMDA) versus non-NMDA receptor/channel activation. Neurosci Lett 366: 320-325.
- De Felice FG, Velasco PT, Lambert MP, Viola K, Fernandez SJ, et al. (2007) Abeta oligomers induce neuronal oxidative stress through an N-methyl-D-aspartate receptor-dependent mechanism that is blocked by the Alzheimer drug memantine. J Biol Chem 282: 11590-11601.
- Shankar GM, Bloodgood BL, Townsend M, Walsh DM, Selkoe DJ, et al. (2007) Natural oligomers of the Alzheimer amyloid-beta protein induce reversible synapse loss by modulating an NMDA-type glutamate receptor-dependent signaling pathway. J Neurosci 27: 2866-2875.
- Kelly BL, Ferreira A (2006) beta-Amyloid-induced dynamin 1 degradation is mediated by N-methyl-D-aspartate receptors in hippocampal neurons. J Biol Chem 281: 28079-28089.
- Li S, Hong S, Shepardson NE, Walsh DM, Shankar GM, et al. (2009) Soluble oligomers of amyloid Beta protein facilitate hippocampal long-term depression by disrupting neuronal glutamate uptake. Neuron 62: 788-801.
- Snyder EM, Nong Y, Almeida CG, Paul S, Moran T, et al. (2005) Regulation of NMDA receptor trafficking by amyloid-beta. Nat Neurosci 8: 1051-1058.
- Dewachter I, Filipkowski RK, Priller C, Ris L, Neyton J, et al. (2009) Deregulation of NMDA-receptor function and down-stream signaling in APP[V717I] transgenic mice. Neurobiol Aging 30: 241-256.
- D'Andrea MR, Nagele RG (2006) Targeting the alpha 7 nicotinic acetylcholine receptor to reduce amyloid accumulation in Alzheimer's disease pyramidal neurons. Curr Pharm Des 12: 677-684.
- Wang HY, Lee DH, D'Andrea MR, Peterson PA, Shank RP, et al. (2000) beta-Amyloid(1-42) binds to alpha7 nicotinic acetylcholine receptor with high affinity. Implications for Alzheimer's disease pathology. J Biol Chem 275: 5626-5632.
- Buckingham SD, Jones AK, Brown LA, Sattelle DB (2009) Nicotinic acetylcholine receptor signalling: roles in Alzheimer's disease and amyloid neuroprotection. Pharmacol Rev 61: 39-61.
- Wevers A, Monteggia L, Nowacki S, Bloch W, Schütz U, et al. (1999) Expression of nicotinic acetylcholine receptor subunits in the cerebral cortex in Alzheimer's disease: histotopographical correlation with amyloid plaques and hyperphosphorylated-tau protein. Eur J Neurosci 11: 2551-2565.
- Bartus RT, Dean RL 3rd, Beer B, Lippa AS (1982) The cholinergic hypothesis of geriatric memory dysfunction. Science 217: 408-414.
- Arneric SP, Holladay M, Williams M (2007) Neuronal nicotinic receptors: a perspective on two decades of drug discovery research. Biochem Pharmacol 74: 1092-1101.
- Schapansky J, Olson K, Van Der Ploeg R, Glazner G (2007) NF-kappaB activated by ER calcium release inhibits Abeta-mediated expression of CHOP protein: enhancement by AD-linked mutant presenilin 1. Exp Neurol 208: 169-176.
- Casley CS, Lakics V, Lee HG, Broad LM, Day TA, et al. (2009) Up-regulation of astrocyte metabotropic glutamate receptor 5 by amyloid-beta peptide. Brain Res.
- Shtifman A, Ward CW, Laver DR, Bannister ML, Lopez JR, et al. (2010) Amyloid-beta protein impairs Ca2+ release and contractility in skeletal muscle. Neurobiol Aging 31: 2080-2090.
- Supnet C, Grant J, Kong H, Westaway D, Mayne M (2006) Amyloid-beta-(1-42) increases ryanodine receptor-3 expression and function in neurons of TgCRND8 mice. J Biol Chem 281: 38440-38447.
- Tu H, Nelson O, Bezprozvanny A, Wang Z, Lee SF, et al. (2006) Presenilins form ER Ca2+ leak channels, a function disrupted by familial Alzheimer's disease-linked mutations. Cell 126: 981-993.
- Nelson O, Tu H, Lei T, Bentahir M, de Strooper B, et al. (2007) Familial Alzheimer disease-linked mutations specifically disrupt Ca2+ leak function of presenilin 1. J Clin Invest 117: 1230-1239.
- Supnet C, Bezprozvanny I (2011) Presenilins as endoplasmic reticulum calcium leak channels and Alzheimer's disease pathogenesis. Sci China Life Sci 54: 744-751.
- Stutzmann GE (2005) Calcium dysregulation, IP3 signaling, and Alzheimer's disease. Neuroscientist 11: 110-115.
- Arispe N, Pollard HB, Rojas E (1993) Giant multilevel cation channels formed by Alzheimer disease amyloid beta-protein [A beta P-(1-40)] in bilayer membranes. Proc Natl Acad Sci U S A 90: 10573-10577.
- Arispe N, Rojas E, Pollard HB (1993) Alzheimer disease amyloid beta protein forms calcium channels in bilayer membranes: blockade by tromethamine and aluminum. Proc Natl Acad Sci U S A 90: 567-571.
- Arispe N, Pollard HB, Rojas E (1994) The ability of amyloid beta-protein [A beta P (1-40)] to form Ca2+ channels provides a mechanism for neuronal death in Alzheimer's disease. Ann N Y Acad Sci 747: 256-266.
- Arispe N (2004) Architecture of the Alzheimer's A beta P ion channel pore. J Membr Biol 197: 33-48.
- Rhee SK, Quist AP, Lal R (1998) Amyloid beta protein-(1-42) forms calcium-permeable, Zn2+-sensitive channel. J Biol Chem 273: 13379-13382.
- Kawahara M, Arispe N, Kuroda Y, Rojas E (1997) Alzheimer's disease amyloid beta-protein forms Zn(2+)-sensitive, cation-selective channels across excised membrane patches from hypothalamic neurons. Biophys J 73: 67-75.
- Lin H, Bhatia R, Lal R (2001) Amyloid beta protein forms ion channels: implications for Alzheimer's disease pathophysiology. FASEB J 15: 2433-2444.
- Quist A, Doudevski I, Lin H, Azimova R, Ng D, et al. (2005) Amyloid ion channels: a common structural link for protein-misfolding disease. Proc Natl Acad Sci U S A 102: 10427-10432.
- Lashuel HA, Hartley D, Petre BM, Walz T, Lansbury PT Jr (2002) Neurodegenerative disease: amyloid pores from pathogenic mutations. Nature 418: 291.
- Inoue S (2008) In situ Abeta pores in AD brain are cylindrical assembly of Abeta protofilaments. Amyloid 15: 223-233.
- Diaz JC, Simakova O, Jacobson KA, Arispe N, Pollard HB (2009) Small molecule blockers of the Alzheimer Abeta calcium channel potently protect neurons from Abeta cytotoxicity. Proc Natl Acad Sci U S A 106: 3348-3353.
- Decout A, Labeur C, Goethals M, Brasseur R, Vandekerckhove J, et al. (1998) Enhanced efficiency of a targeted fusogenic peptide. Biochim Biophys Acta 1372: 102-116.
- Terzi E, Holzemann G, Seelig J (1995) Self-association of beta-amyloid peptide (1-40) in solution and binding to lipid membranes. J Mol Biol 252: 633-642.
- Cribbs DH, Pike CJ, Weinstein SL, Velazquez P, Cotman CW (1997) All-D-enantiomers of beta-amyloid exhibit similar biological properties to all-L-beta-amyloids. J Biol Chem 272: 7431-7436.
- Avdulov NA, Chochina SV, Igbavboa U, Warden CS, Vassiliev AV, et al. (1997) Lipid binding to amyloid beta-peptide aggregates: preferential binding of cholesterol as compared with phosphatidylcholine and fatty acids. J Neurochem 69: 1746-1752.
- McLaurin J, Franklin T, Chakrabartty A, Fraser PE (1998) Phosphatidylinositol and inositol involvement in Alzheimer amyloid-beta fibril growth and arrest. J Mol Biol 278: 183-194.
- Muller WE, Koch S, Eckert A, Hartmann H, Scheuer K (1995) beta-Amyloid peptide decreases membrane fluidity. Brain Res 674: 133-136.
- Grimm MO, Tschäpe JA, Grimm HS, Zinser EG, Hartmann T (2006) Altered membrane fluidity and lipid raft composition in presenilin-deficient cells. Acta Neurol Scand Suppl 185: 27-32.
- Bernardi P, Krauskopf A, Basso E, Petronilli V, Blachly-Dyson E, et al. (2006) The mitochondrial permeability transition from in vitro artifact to disease target. FEBS J 273: 2077-2099.
- Brustovetsky N, LaFrance R, Purl KJ, Brustovetsky T, Keene CD, et al. (2005) Age-dependent changes in the calcium sensitivity of striatal mitochondria in mouse models of Huntington's Disease. J Neurochem 93: 1361-1370.
- Lovell MA, Xie C, Markesbery WR (2001) Acrolein is increased in Alzheimer's disease brain and is toxic to primary hippocampal cultures. Neurobiol Aging 22: 187-194.
- Manczak M, Anekonda TS, Henson E, Park BS, Quinn J, et al. (2006) Mitochondria are a direct site of A beta accumulation in Alzheimer's disease neurons: implications for free radical generation and oxidative damage in disease progression. Hum Mol Genet 15: 1437-1449.
- Apelt J, Bigl M, Wunderlich P, Schliebs R (2004) Aging-related increase in oxidative stress correlates with developmental pattern of beta-secretase activity and beta-amyloid plaque formation in transgenic Tg2576 mice with Alzheimer-like pathology. Int J Dev Neurosci 22: 475-484.
- Simpson JE, Ince PG, Haynes LJ, Theaker R, Gelsthorpe C, et al. (2010) Population variation in oxidative stress and astrocyte DNA damage in relation to Alzheimer-type pathology in the ageing brain. Neuropathol Appl Neurobiol 36: 25-40.
- Tamagno E, Guglielmotto M, Aragno M, Borghi R, Autelli R, et al. (2008) Oxidative stress activates a positive feedback between the gamma- and beta-secretase cleavages of the beta-amyloid precursor protein. J Neurochem 104: 683-695.
- Tong Y, Zhou W, Fung V, Christensen MA, Qing H, et al. (2005) Oxidative stress potentiates BACE1 gene expression and Abeta generation. J Neural Transm 112: 455-469.
- Akiyama H, Barger S, Barnum S, Bradt B, Bauer J, et al. (2000) Inflammation and Alzheimer's disease. Neurobiol Aging 21: 383-421.
- Giovannini MG, Scali C, Prosperi C, Bellucci A, Vannucchi MG, et al. (2002) Beta-amyloid-induced inflammation and cholinergic hypofunction in the rat brain in vivo: involvement of the p38MAPK pathway. Neurobiol Dis 11: 257-274.
- Salminen A, Ojala J, Kauppinen A, Kaarniranta K, Suuronen T (2009) Inflammation in Alzheimer's disease: amyloid-beta oligomers trigger innate immunity defence via pattern recognition receptors. Prog Neurobiol 87: 181-194.
- Walter S, Letiembre M, Liu Y, Heine H, Penke B, et al. (2007) Role of the toll-like receptor 4 in neuroinflammation in Alzheimer's disease. Cell Physiol Biochem 20: 947-956.
- Kauppinen TM, Suh SW, Higashi Y, Berman AE, Escartin C, et al. (2011) Poly(ADP-ribose)polymerase-1 modulates microglial responses to amyloid beta. J Neuroinflammation 8: 152.
- White JA, Manelli AM, Holmberg KH, Van Eldik LJ, Ladu MJ (2005) Differential effects of oligomeric and fibrillar amyloid-beta 1-42 on astrocyte-mediated inflammation. Neurobiol Dis 18: 459-465.
- DaRocha-Souto B, Scotton TC, Coma M, Serrano-Pozo A, Hashimoto T, et al. (2011) Brain oligomeric beta-amyloid but not total amyloid plaque burden correlates with neuronal loss and astrocyte inflammatory response in amyloid precursor protein/tau transgenic mice. J Neuropathol Exp Neurol 70: 360-376.
- Garwood CJ, Pooler AM, Atherton J, Hanger DP, Noble W (2011) Astrocytes are important mediators of Abeta-induced neurotoxicity and tau phosphorylation in primary culture. Cell Death Dis 2: e167.
- Lee JW, Lee YK, Yuk DY, Choi DY, Ban SB, et al. (2008) Neuro-inflammation induced by lipopolysaccharide causes cognitive impairment through enhancement of beta-amyloid generation. J Neuroinflammation 5: 37.
- Sheng JG, Bora SH, Xu G, Borchelt DR, Price DL, et al. (2003) Lipopolysaccharide-induced-neuroinflammation increases intracellular accumulation of amyloid precursor protein and amyloid beta peptide in APPswe transgenic mice. Neurobiol Dis 14: 133-145.
- Heneka MT, O'Banion MK, Terwel D, Kummer MP (2010) Neuroinflammatory processes in Alzheimer's disease. J Neural Transm 117: 919-947.
- Agostinho P, Cunha RA, Oliveira C (2010) Neuroinflammation, oxidative stress and the pathogenesis of Alzheimer's disease. Curr Pharm Des 16: 2766-2778.
- Candore G, Bulati M, Caruso C, Castiglia L, Colonna-Romano G, et al. (2010) Inflammation, cytokines, immune response, apolipoprotein E, cholesterol, and oxidative stress in Alzheimer disease: therapeutic implications. Rejuvenation Res 13: 301-313.
- Logeat F, Bessia C, Brou C, LeBail O, Jarriault S, et al. (1998) The Notch1 receptor is cleaved constitutively by a furin-like convertase. Proc Natl Acad Sci U S A 95: 8108-8112.
- Brou C, Logeat F, Gupta N, Bessia C, LeBail O, et al. (2000) A novel proteolytic cleavage involved in Notch signaling: the role of the disintegrin-metalloprotease TACE. Mol Cell 5: 207-216.
- Mumm JS, Schroeter EH, Saxena MT, Griesemer A, Tian X, et al. (2000) A ligand-induced extracellular cleavage regulates gamma-secretase-like proteolytic activation of Notch1. Mol Cell 5: 197-206.
- Bozkulak EC, Weinmaster G (2009) Selective use of ADAM10 and ADAM17 in activation of Notch1 signaling. Mol Cell Biol 29: 5679-5695.
- Weber S, Niessen MT, Prox J, Lullmann-Rauch R, Schmitz A, et al. (2011) The disintegrin/metalloproteinase Adam10 is essential for epidermal integrity and Notch-mediated signaling. Development 138: 495-505.
- Struhl G, Greenwald I (19990 Presenilin is required for activity and nuclear access of Notch in Drosophila. Nature 398: 522-525.
- De Strooper B, Annaert W, Cupers P, Saftig P, Craessaerts K, et al. (1999) A presenilin-1-dependent gamma-secretase-like protease mediates release of Notch intracellular domain. Nature 398: 518-522.
- Ray WJ, Yao M, Mumm J, Schroeter EH, Saftig P, et al. (1999) Cell surface presenilin-1 participates in the gamma-secretase-like proteolysis of Notch. J Biol Chem 274: 36801-36807.
- Schroeter EH, Kisslinger JA, Kopan R (1998) Notch-1 signalling requires ligand-induced proteolytic release of intracellular domain. Nature 393: 382-386.
- Kageyama R, Ohtsuka T (1999) The Notch-Hes pathway in mammalian neural development. Cell Res 9: 179-188.
- Fortini ME (2009) Notch signaling: the core pathway and its posttranslational regulation. Dev Cell 16: 633-647.
- Ables JL, Breunig JJ, Eisch AJ, Rakic P (2011) Not(ch) just development: Notch signalling in the adult brain. Nat Rev Neurosci 12: 269-283.
- Sestan N, Artavanis-Tsakonas S, Rakic P (1999) Contact-dependent inhibition of cortical neurite growth mediated by notch signaling. Science 286: 741-746.
- Hitoshi S, Alexson T, Tropepe V, Donoviel D, Elia AJ, et al. (2002) Notch pathway molecules are essential for the maintenance, but not the generation, of mammalian neural stem cells. Genes Dev 16: 846-858.
- Poirazi P, Mel BW (2001) Impact of active dendrites and structural plasticity on the memory capacity of neural tissue. Neuron 29: 779-796.
- Shors TJ, Miesegaes G, Beylin A, Zhao M, Rydel T, et al. (2001) Neurogenesis in the adult is involved in the formation of trace memories. Nature 410: 372-376.
- Sjolund J, Manetopoulos C, Stockhausen MT, Axelson H (2005) The Notch pathway in cancer: differentiation gone awry. Eur J Cancer 41: 2620-2629.
- Roy M, Pear WS, Aster JC (2007) The multifaceted role of Notch in cancer. Curr Opin Genet Dev 17: 52-59.
- Joutel A, Corpechot C, Ducros A, Vahedi K, Chabriat H, et al. (1996) Notch3 mutations in CADASIL, a hereditary adult-onset condition causing stroke and dementia. Nature 383: 707-710.
- Li L, Krantz ID, Deng Y, Genin A, Banta AB, et al. (1997) Alagille syndrome is caused by mutations in human Jagged1, which encodes a ligand for Notch1. Nat Genet 16: 243-251.
- Oda T, Elkahloun AG, Pike BL, Okajima K, Krantz ID, et al. (1997) Mutations in the human Jagged1 gene are responsible for Alagille syndrome. Nat Genet 16: 235-242.
- Isidor B, Lindenbaum P, Pichon O, Bezieau S, Dina C, et al. (2011) Truncating mutations in the last exon of NOTCH2 cause a rare skeletal disorder with osteoporosis. Nat Genet 43: 306-308.
- Simpson MA, Irving MD, Asilmaz E, Gray MJ, Dafou D, et al. (2011). Mutations in NOTCH2 cause Hajdu-Cheney syndrome, a disorder of severe and progressive bone loss. Nat Genet 43: 303-305.
- Jurynczyk M, Selmaj K (2010) Notch: a new player in MS mechanisms. J Neuroimmunol 218: 3-11.
- Fischer DF, van Dijk R, Sluijs JA, Nair SM, Racchi M, et al. (2005) Activation of the Notch pathway in Down syndrome: cross-talk of Notch and APP. FASEB J 19: 1451-1458.
- Berezovska O, Xia MQ, Hyman BT (1998) Notch is expressed in adult brain, is coexpressed with presenilin-1, and is altered in Alzheimer disease. J Neuropathol Exp Neurol 57: 738-745.
- Costa RM, Honjo T, Silva AJ (2003) Learning and memory deficits in Notch mutant mice. Curr Biol 13: 1348-1354.
- Selkoe D, Kopan R (2003) Notch and Presenilin: regulated intramembrane proteolysis links development and degeneration. Annu Rev Neurosci 26: 565-597.
- Ray WJ, Yao M, Nowotny P, Mumm J, Zhang W, et al. (1999) Evidence for a physical interaction between presenilin and Notch. Proc Natl Acad Sci USA 96: 3263-3268.
- Fassa A, Mehta P, Efthimiopoulos S (2005) Notch 1 interacts with the amyloid precursor protein in a Numb-independent manner. J Neurosci Res 82: 214-224.
- Roncarati R, Sestan N, Scheinfeld MH, Berechid BE, Lopez PA, et al. (2002) The gamma-secretase-generated intracellular domain of beta-amyloid precursor protein binds Numb and inhibits Notch signaling. Proc Natl Acad Sci USA 99: 7102-7107.
- Veeraraghavalu K, Choi SH, Zhang X, Sisodia SS (2010) Presenilin 1 mutants impair the self-renewal and differentiation of adult murine subventricular zone-neuronal progenitors via cell-autonomous mechanisms involving notch signaling. J Neurosci 30: 6903-6915.
- Grundke-Iqbal, I, Iqbal K, Tung YC, Quinlan M, Wisniewski HM, et al. (1986) Abnormal phosphorylation of the microtubule-associated protein tau (tau) in Alzheimer cytoskeletal pathology. Proc Natl Acad Sci USA 83: 4913-4917.
- Grundke-Iqbal I, Iqbal K, Quinlan M, Tung YC, Zaidi MS, et al. (1986) Microtubule-associated protein tau. A component of Alzheimer paired helical filaments. J Biol Chem 261: 6084-6089.
- Lee VM, Balin BJ, Otvos L Jr, Trojanowski JQ (1991) A68: a major subunit of paired helical filaments and derivatized forms of normal Tau. Science 251: 675-678.
- Goedert M, Spillantini MG, Cairns NJ, Crowther RA (1992) Tau proteins of Alzheimer paired helical filaments: abnormal phosphorylation of all six brain isoforms. Neuron 8: 159-168.
- Binder LI, Frankfurter A, Rebhun LI (1985) The distribution of tau in the mammalian central nervous system. J Cell Biol 101: 1371-1378.
- Butner KA, Kirschner MW (1991) Tau protein binds to microtubules through a flexible array of distributed weak sites. J Cell Biol 115: 717-730.
- Weingarten MD, Lockwood AH, Hwo SY, Kirschner MW (1975) A protein factor essential for microtubule assembly. Proc Natl Acad Sci USA 72: 1858-1862.
- Goedert M, Spillantini MG, Jakes R, Rutherford D, Crowther RA (1989) Multiple isoforms of human microtubule-associated protein tau: sequences and localization in neurofibrillary tangles of Alzheimer's disease. Neuron 3: 519-526.
- Lindwall G, Cole RD (1984) Phosphorylation affects the ability of tau protein to promote microtubule assembly. J Biol Chem 259: 5301-5305.
- Alonso AC, Zaidi T, Grundke-Iqbal I, Iqbal K (1994) Role of abnormally phosphorylated tau in the breakdown of microtubules in Alzheimer disease. Proc Natl Acad Sci U S A 91: 5562-5566.
- Dickson DW (1998) Pick's disease: a modern approach. Brain Pathol 8: 339-354.
- Bergeron C, Davis A, Lang AE (1998) Corticobasal ganglionic degeneration and progressive supranuclear palsy presenting with cognitive decline. Brain Pathol 8: 355-365.
- Higuchi M, Trojanowski JQ, Lee VMY (2002) Tau Protein and Tauopathy. In Neuropsychopharmacology: The Fifth Generation of Progress. K. L. Davis, D. Charney, J. T. Coyle, and C. Nemeroff, editors, American College of Neuropsychopharmacology.
- Hanger DP, Betts JC, Loviny TL, Blackstock WP, Anderton BH (1998) New phosphorylation sites identified in hyperphosphorylated tau (paired helical filament-tau) from Alzheimer's disease brain using nanoelectrospray mass spectrometry. J Neurochem 71: 2465-2476.
- Morishima-Kawashima M, Hasegawa M, Takio K, Suzuki M, Yoshida H, et al. (1995) Proline-directed and non-proline-directed phosphorylation of PHF-tau. J Biol Chem 270: 823-829.
- Billingsley ML, Kincaid RL (1997) Regulated phosphorylation and dephosphorylation of tau protein: effects on microtubule interaction, intracellular trafficking and neurodegeneration. Biochem J 323: 577-591.
- Wang JZ, Wu Q, Smith A, Grundke-Iqbal I, Iqbal K (1998) Tau is phosphorylated by GSK-3 at several sites found in Alzheimer disease and its biological activity markedly inhibited only after it is prephosphorylated by A-kinase. FEBS Lett 436: 28-34.
- Liu SJ, Zhang JY, Li HL, Fang ZY, Wang Q, et al. (2004) Tau becomes a more favorable substrate for GSK-3 when it is prephosphorylated by PKA in rat brain. J Biol Chem 279: 50078-50088.
- Cuchillo-Ibanez I, Seereeram A, Byers HL, Leung KY, Ward MA, et al. (2008) Phosphorylation of tau regulates its axonal transport by controlling its binding to kinesin. FASEB J 22: 3186-3195.
- Rankin CA, Sun Q, Gamblin TC (2007) Tau phosphorylation by GSK-3beta promotes tangle-like filament morphology. Mol Neurodegener 2: 12.
- Lucas JJ, Hernández F, Gomez-Ramos P, Moran MA, Hen R, et al. (2001) Decreased nuclear beta-catenin, tau hyperphosphorylation and neurodegeneration in GSK-3beta conditional transgenic mice. EMBO J 20: 27-39.
- Wagner U, Utton M, Gallo JM, Miller CC (1996) Cellular phosphorylation of tau by GSK-3 beta influences tau binding to microtubules and microtubule organisation. J Cell Sci 109: 1537-1543.
- Hong M, Chen DC, Klein PS, Lee VM (1997) Lithium reduces tau phosphorylation by inhibition of glycogen synthase kinase-3. J Biol Chem 272: 25326-25332.
- Perez M, Hernández F, Lim F, Díaz-Nido J, Avila J (2003) Chronic lithium treatment decreases mutant tau protein aggregation in a transgenic mouse model. J Alzheimers Dis 5: 301-308.
- Scott CW, Spreen RC, Herman JL, Chow FP, Davison MD, et al. (1993) Phosphorylation of recombinant tau by cAMP-dependent protein kinase. Identification of phosphorylation sites and effect on microtubule assembly. J Biol Chem 268: 1166-1173.
- Black AR, Jensen D, Lin SY, Azizkhan JC (1999) Growth/cell cycle regulation of Sp1 phosphorylation. J Biol Chem 274: 1207-1215.
- Li G, Yin H, Kuret J (2004) Casein kinase 1 delta phosphorylates tau and disrupts its binding to microtubules. J Biol Chem 279: 15938-15945.
- Hanger DP, Byers HL, Wray S, Leung KY, Saxton MJ, et al. (2007) Novel phosphorylation sites in tau from Alzheimer brain support a role for casein kinase 1 in disease pathogenesis. J Biol Chem 282: 23645-23654.
- Lew J, Huang QQ, Qi Z, Winkfein RJ, Aebersold R, et al. (1994) A brain-specific activator of cyclin-dependent kinase 5. Nature 371: 423-426.
- Tsai LH, Takahashi T, Caviness VS Jr, Harlow E (1993) Activity and expression pattern of cyclin-dependent kinase 5 in the embryonic mouse nervous system. Development 119: 1029-1040.
- Woodgett JR (1990) Molecular cloning and expression of glycogen synthase kinase-3/factor A. EMBO J 9: 2431-2438.
- Pei JJ, Grundke-Iqbal I, Iqbal K, Bogdanovic N, Winblad B, et al. (1998) Accumulation of cyclin-dependent kinase 5 (cdk5) in neurons with early stages of Alzheimer's disease neurofibrillary degeneration. Brain Res 797: 267-277.
- Pei JJ, Braak E, Braak H, Grundke-Iqbal I, Iqbal K, et al. (1999) Distribution of active glycogen synthase kinase 3beta (GSK-3beta) in brains staged for Alzheimer disease neurofibrillary changes. J Neuropathol Exp Neurol 58: 1010-1019.
- Piedrahita D, Hernandez I, López-Tobon A, Fedorov D, Obara B, et al. (2010) Silencing of CDK5 reduces neurofibrillary tangles in transgenic alzheimer's mice. J Neurosci 30: 13966-13976.
- Cruz JC, Tseng HC, Goldman JA, Shih H, Tsai LH (2003) Aberrant Cdk5 activation by p25 triggers pathological events leading to neurodegeneration and neurofibrillary tangles. Neuron 40: 471-483.
- Zheng, Y. L., S. Kesavapany, M. Gravell, R. S. Hamilton, M. Schubert, N. Amin, W. Albers, P. Grant, and H. C. Pant. 2005. A Cdk5 inhibitory peptide reduces tau hyperphosphorylation and apoptosis in neurons. EMBO J 24: 209-220.
- Patrick GN, Zukerberg L, Nikolic M, de la Monte S, Dikkes P, et al. (1999) Conversion of p35 to p25 deregulates Cdk5 activity and promotes neurodegeneration. Nature 402: 615-622.
- Ferrer I, Blanco R, Carmona M, Ribera R, Goutan E, et al. (2001) Phosphorylated map kinase (ERK1, ERK2) expression is associated with early tau deposition in neurones and glial cells, but not with increased nuclear DNA vulnerability and cell death, in Alzheimer disease, Pick's disease, progressive supranuclear palsy and corticobasal degeneration. Brain Pathol 11: 144-158.
- Sawamura N, Gong JS, Chang TY, Yanagisawa K, Michikawa M (2003) Promotion of tau phosphorylation by MAP kinase Erk1/2 is accompanied by reduced cholesterol level in detergent-insoluble membrane fraction in Niemann-Pick C1-deficient cells. J Neurochem 84: 1086-1096.
- An WL, Cowburn RF, Li L, Braak H, Alafuzoff I, et al. (2003) Up-regulation of phosphorylated/activated p70 S6 kinase and its relationship to neurofibrillary pathology in Alzheimer's disease. Am J Pathol 163: 591-607.
- Atzori C, Ghetti B, Piva R, Srinivasan AN, Zolo P, et al. (2001) Activation of the JNK/p38 pathway occurs in diseases characterized by tau protein pathology and is related to tau phosphorylation but not to apoptosis. J Neuropathol Exp Neurol 60: 1190-1197.
- Pei JJ, Braak E, Braak H, Grundke-Iqbal I, Iqbal K, et al. (2001) Localization of active forms of C-jun kinase (JNK) and p38 kinase in Alzheimer's disease brains at different stages of neurofibrillary degeneration. J Alzheimers Dis 3: 41-48.
- Drewes G, Lichtenberg-Kraag B, Döring F, Mandelkow EM, Biernat J, et al. (1992) Mitogen activated protein (MAP) kinase transforms tau protein into an Alzheimer-like state. EMBO J 11: 2131-2138.
- Liu F, Liang Z, Wegiel J, Hwang YW, Iqbal K, et al. (2008) Overexpression of Dyrk1A contributes to neurofibrillary degeneration in Down syndrome. FASEB J 22: 3224-3233.
- Woods YL, Cohen P, Becker W, Jakes R, Goedert M, et al. (2001) The kinase DYRK phosphorylates protein-synthesis initiation factor eIF2Bepsilon at Ser539 and the microtubule-associated protein tau at Thr212: potential role for DYRK as a glycogen synthase kinase 3-priming kinase. Biochem J 355: 609-615.
- Liu F, Grundke-Iqbal I, Iqbal K, Gong CX (2005) Contributions of protein phosphatases PP1, PP2A, PP2B and PP5 to the regulation of tau phosphorylation. Eur J Neurosci 22: 1942-1950.
- Oliver CJ, Shenolikar S (1998) Physiologic importance of protein phosphatase inhibitors. Front Biosci 3: D961-972.
- Kins S, Kurosinski P, Nitsch RM, Gotz J (2003) Activation of the ERK and JNK signaling pathways caused by neuron-specific inhibition of PP2A in transgenic mice. Am J Pathol 163: 833-843.
- Vogelsberg-Ragaglia V, Schuck T, Trojanowski JQ, Lee VM (2001) PP2A mRNA expression is quantitatively decreased in Alzheimer's disease hippocampus. Exp Neurol 168: 402-412.
- Gong CX, Shaikh S, Wang JZ, Zaidi T, Grundke-Iqbal I, et al. (1995) Phosphatase activity toward abnormally phosphorylated tau: decrease in Alzheimer disease brain. J Neurochem 65: 732-738.
- Giri RK, Selvaraj SK, Kalra VK (2003) Amyloid peptide-induced cytokine and chemokine expression in THP-1 monocytes is blocked by small inhibitory RNA duplexes for early growth response-1 messenger RNA. J Immunol 170: 5281-5294.
- Giri RK, Rajagopal V, Kalra VK (2004) Curcumin, the active constituent of turmeric, inhibits amyloid peptide-induced cytochemokine gene expression and CCR5-mediated chemotaxis of THP-1 monocytes by modulating early growth response-1 transcription factor. J Neurochem 91: 1199-1210.
- Lu Y, Li T, Qureshi HY, Han D, Paudel HK (2011) Early growth response 1 (Egr-1) regulates phosphorylation of microtubule-associated protein tau in mammalian brain. J Biol Chem 286: 20569-20581.
- Jicha GA, Berenfeld B, Davies P (1999) Sequence requirements for formation of conformational variants of tau similar to those found in Alzheimer's disease. J Neurosci Res 55: 713-723.
- Jicha GA, Rockwood JM, Berenfeld B, Hutton M, Davies P (1999) Altered conformation of recombinant frontotemporal dementia-17 mutant tau proteins. Neurosci Lett 260: 153-156.
- Cotman CW, Poon WW, Rissman RA, Blurton-Jones M (2005) The role of caspase cleavage of tau in Alzheimer disease neuropathology. J Neuropathol Exp Neurol 64: 104-112.
- Gamblin TC, Chen F, Zambrano A, Abraha A, Lagalwar S, et al. (2003) Caspase cleavage of tau: linking amyloid and neurofibrillary tangles in Alzheimer's disease. Proc Natl Acad Sci U S A 100: 10032-10037.
- Zilka N, Filipcik P, Koson P, Fialova L, Skrabana R, et al. (2006) Truncated tau from sporadic Alzheimer's disease suffices to drive neurofibrillary degeneration in vivo. FEBS Lett 580: 3582-3588.
- Wang JZ, Grundke-Iqbal I, Iqbal K (1996) Restoration of biological activity of Alzheimer abnormally phosphorylated tau by dephosphorylation with protein phosphatase-2A, -2B and -1. Brain Res Mol Brain Res 38: 200-208.
- Yang LS, Ksiezak-Reding H (1995) Calpain-induced proteolysis of normal human tau and tau associated with paired helical filaments. Eur J Biochem 233: 9-17.
- Poppek D, Keck S, Ermak G, Jung T, Stolzing A, et al. (2006) Phosphorylation inhibits turnover of the tau protein by the proteasome: influence of RCAN1 and oxidative stress. Biochem J 400: 511-520.
- Khatoon S, Grundke-Iqbal I, Iqbal K (1992) Brain levels of microtubule-associated protein tau are elevated in Alzheimer's disease: a radioimmuno-slot-blot assay for nanograms of the protein. J Neurochem 59: 750-753.
- Iqbal K, Liu F, Gong CX, Alonso Adel C, Grundke-Iqbal I (2009) Mechanisms of tau-induced neurodegeneration. Acta Neuropathol 118: 53-69.
- Liazoghli D, Perreault S, Micheva KD, Desjardins M, Leclerc N (2005) Fragmentation of the Golgi apparatus induced by the overexpression of wild-type and mutant human tau forms in neurons. Am J Pathol 166: 1499-1514.
- Yoshiyama Y, Higuchi M, Zhang B, Huang SM, Iwata N, et al. (2007) Synapse loss and microglial activation precede tangles in a P301S tauopathy mouse model. Neuron 53: 337-351.
- Kim I, Xu W, Reed JC (2008) Cell death and endoplasmic reticulum stress: disease relevance and therapeutic opportunities. Nat Rev Drug Discov 7: 1013-1030.
- Terwel D, Muyllaert D, Dewachter I, Borghgraef P, Croes S, et al. (2008) Amyloid activates GSK-3beta to aggravate neuronal tauopathy in bigenic mice. Am J Pathol 172: 786-798.
- Baki L, Shioi J, Wen P, Shao Z, Schwarzman A, et al. (2004) PS1 activates PI3K thus inhibiting GSK-3 activity and tau overphosphorylation: effects of FAD mutations. EMBO J 23: 2586-2596.
- Lewis J, Dickson DW, Lin WL, Chisholm L, Corral A, et al. (2001) Enhanced neurofibrillary degeneration in transgenic mice expressing mutant tau and APP. Science 293: 1487-1491.
- Gotz J, Chen F, van Dorpe J, Nitsch RM (2001) Formation of neurofibrillary tangles in P301l tau transgenic mice induced by Abeta 42 fibrils. Science 293: 1491-1495.
- Oddo S, Billings L, Kesslak JP, Cribbs DH, LaFerla FM (2004) Abeta immunotherapy leads to clearance of early, but not late, hyperphosphorylated tau aggregates via the proteasome. Neuron 43: 321-332.
- Ribe EM, Perez M, Puig B, Gich I, Lim F, et al. (2005) Accelerated amyloid deposition, neurofibrillary degeneration and neuronal loss in double mutant APP/tau transgenic mice. Neurobiol Dis 20: 814-822.
- Roberson ED, Scearce-Levie K, Palop JJ, Yan F, Cheng IH, et al. (2007) Reducing endogenous tau ameliorates amyloid beta-induced deficits in an Alzheimer's disease mouse model. Science 316: 750-754.
- Ittner LM, Ke YD, Delerue F, Bi M, Gladbach A, et al. (2010) Dendritic function of tau mediates amyloid-beta toxicity in Alzheimer's disease mouse models. Cell 142: 387-397.
- Rapoport M, Dawson HN, Binder LI, Vitek MP, Ferreira A (2002) Tau is essential to beta -amyloid-induced neurotoxicity. Proc Natl Acad Sci U S A 99: 6364-6369.
- Grimm MO, Grimm HS, Hartmann T (2007) Amyloid beta as a regulator of lipid homeostasis. Trends Mol Med 13: 337-344.
- Zinser EG, Hartmann T, Grimm MO (2007) Amyloid beta-protein and lipid metabolism. Biochim Biophys Acta 1768: 1991-2001.
- Giuffrida ML, Caraci F, De Bona P, Pappalardo G, Nicoletti F, et al. (2010) The monomer state of beta-amyloid: where the Alzheimer's disease protein meets physiology. Rev Neurosci 21: 83-93.
- Kim D, Tsai LH (2009) Bridging physiology and pathology in AD. Cell 137: 997-1000.
- Avila J, Lucas JJ, Perez M, Hernandez F (2004) Role of tau protein in both physiological and pathological conditions. Physiol Rev 84: 361-384.
- von Rotz RC, Kohli BM, Bosset J, Meier M, Suzuki T, et al. (2004) The APP intracellular domain forms nuclear multiprotein complexes and regulates the transcription of its own precursor. J Cell Sci 117: 4435-4448.
- Hebert SS, Serneels L, Tolia A, Craessaerts K, Derks C, et al. (2006) Regulated intramembrane proteolysis of amyloid precursor protein and regulation of expression of putative target genes. EMBO Rep 7: 739-745.
- Cao X, Südhof TC (2001) A transcriptionally [correction of transcriptively] active complex of APP with Fe65 and histone acetyltransferase Tip60. Science 293: 115-120.
- Grimm MO, Grösgen S, Rothhaar TL, Burg VK, Hundsdörferr B, et al. (2011) Intracellular APP Domain Regulates Serine-Palmitoyl-CoA Transferase Expression and Is Affected in Alzheimer's Disease. Int J Alzheimers Dis 2011: 695413.
- Grimm MO, Kuchenbecker J, Rothhaar TL, Grösgen S, Hundsdörferr B, et al. (2011) Plasmalogen synthesis is regulated via alkyl-dihydroxyacetonephosphate-synthase by amyloid precursor protein processing and is affected in Alzheimer's disease. J Neurochem 116: 916-925.
--